Silver Peak |
|
Nevada, USA |
Main commodities:
Li
|
|
 |
|
 |
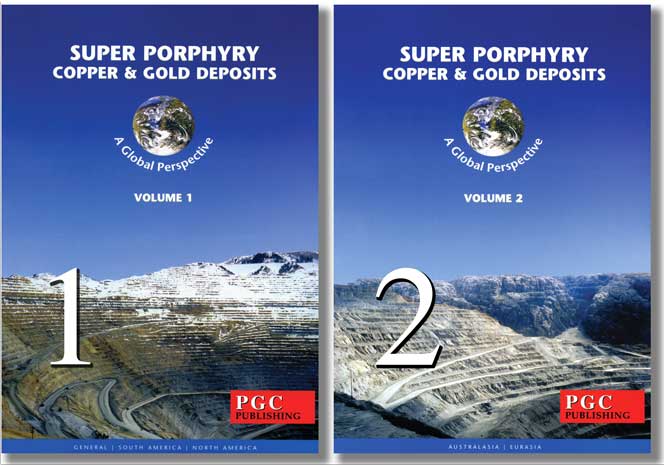 |
Super Porphyry Cu and Au

|
IOCG Deposits - 70 papers
|
All papers now Open Access.
Available as Full Text for direct download or on request. |
|
 |
The Silver Peak Lithium Operations are situated in Clayton Valley, a flat, arid valley, covered with dry playa lake beds, and bounded by ridges that are up to a 1000 m taller, located ~50 km southwest of Tonopah, in Esmeralda County, Nevada, United States (#Location: 37° 46' 23"N, 117° 34' 26"W).
Sodium and potassium brines were first discovered in Clayton Valley in 1912, although the first mining and milling water rights were not secured until 1936, when they were granted to Leprechaun Mining. In the 1950s, that same company discovered lithium in groundwater. In 1964, the Foote Mineral Company acquired land in Clayton Valley and in 1966 commenced in situ mining of lithium, followed in 1967 by the first lithium carbonate production. In 1988, Foote Mining was taken over by Cyprus Amax Minerals and became Cyprus Foote Mineral Co.. The latter subsidiary was subsequently acquired by the German company Chemetall in 1988 to become Chemetall Foote Corp., which was purchased by Rockwood Specialties Group in 2004. In 2015, the NYSE listed Albemarle Corp. bought Rockwood Lithium, Inc. and continues to mine at Silver Peak at a rate of ~5000 to 6000 tpa of Lithium Carbonate Equivalent, with plans to double annual output progressively from 2024.
The lithium brine production facility at Silver Peak has been operated on a continuous basis since the mid-1960s. Production has been from a complex array of wells extracting lithium bearing brine from six different Pleistocene age aquifer systems that have been sequentially brought online over that period. The six aquifer systems, from lowest to uppermost, as described in more detail below, are known as the
Lower Gravel Aquifer (LGA),
Lower Ash System (LAS),
Main Ash Aquifer (MAA),
Marginal Gravel Aquifer (MGA),
Salt Aquifer System (SAS) and
Tufa Aquifer System (TAS).
Whilst all of these aquifers have played important roles in the operation, the MAA is the most developed and extensively exploited.
As the MAA was the primary aquifer system developed over the first half of the mine's history, it has been assumed that the lithium concentration decline/regression trend was predominantly represented by that measured from the MAA, and that the other aquifers only provided a subordinate influence on the lithium concentration. The composite lithium concentration decline/regression trend line developed from this historical data, has been used to project out ~15 years to estimate the lithium concentrations based on similar production rates from the well field. This has proved to be reasonably accurate in providing conservative estimates of the longevity of the in situ mining operation before the economic lithium concentration limit was reached from the brine production. As new aquifer systems were discovered and exploited, the number of wells developed in the MAA began to decline, resulting in a less accurate ore reserve calculation each time. By 2008, only 42% (16) of the wells in the well field were producing from the MAA, whilst MGA, LAS and LGA also generated 42% of the wellfield wells during that same period.
Lithium bearing brines are pumped from the host aquifers via extraction wells, and into evaporation and concentration ponds, before being 'scraped up' and passed through leaching plants, a potash plant and a drying plant, and converted into lithium carbonate at Silver Peak.
In 2024, Silver Peak was, and had been the only operating lithium mine in the US, since possibly the late 1980s.
Regional Setting
The Clayton Valley lies within the Basin and Range Province of western North America, which extends from Canada through the western United States and across much of northern Mexico, encompassing virtually all of Nevada. This province is characterised by block faulting caused by extension and subsequent thinning of the earth’s crust. Particularly in Nevada, this extensional faulting forms NE-SW oriented ridges and valleys, and is responsible for the overall horst and graben structure of Clayton Valley. This period of vertical displacement followed an earlier horizontal extension characterised by listric faulting and metamorphic core complexes.
The timing of major extension varies throughout the province. In eastern Nevada, strongly extensional terrains formed between 34 and 23 Ma in the Oligocene when mountain blocks were tilted and rose along major and minor faults relative to valley blocks, which dropped and became the focal locations for enhanced accumulation of sediments derived from the surrounding mountains. Closed basins, like Clayton Valley, accumulated clastic sediments and evaporites. The Basin and Range Province is also characterised by volcanic activity promoted by the crustal thinning and deep faulting that influenced the rise of magma.
In southern Nevada, basin and range development was also influenced by the dextral Walker Lane Fault Zone, a major transfer structure that accommodates inland displacement related to translation along the margin between the Pacific and North American plates. The latter is marked by the major dextral San Andreas Fault. This transfer results in a corridor of NW-SE transcurrent faults that are estimated to account for between 20 and 25% of the relative motion between the two plates. As a consequence of being proximal to this transition zone, Clayton Valley and areas to the northwest and southeast are located in a more complex zone of deformation and faulting compared to much of the rest of the Basin and Range Province.
Geology
The oldest basement rocks in the vicinity of Clayton Valley are of Neoproterozoic age, conformably overlain by Cambrian and Ordovician lithologies, followed by younger Palaeozoic marine sedimentary rocks, and cut by Jurassic to Tertiary granitic intrusives (Davis et al., 1986). Tertiary volcanic rocks, mainly of rhyolitic composition, emanated from two volcanic centres. The first of these, Montezuma Peak, ~10 km to the east of the Clayton Valley, was active as long as 17 m.y. ago in the Miocene, whilst the younger Silver Peak Centre, a similar distance to the west, was principally active from 6 to 4.8 Ma, from the Late Miocne to Pliocene. Tertiary sedimentary rocks are exposed on the rims of the Clayton Valley, to the west in the Silver Peak Range, the north in the Weepah Hills and as a serties of low hills to the east. All are included in the Esmeralda Formation and comprise sandstone, shale, marl, breccia and conglomerate. These sedimentary rocks are intercalated with volcanic lithologies and were deposited in several Miocene-era basins (Davis et al., 1986).
The majority of basin down drop and displacement is inferred to have occurred across the NNE-SSW Angel Island and NE-SW Paymaster Canyon faults that form the southeastern edge of the basin (Zampirro 2004). The same author also suggests these faults are a barrier to flow into the basin, and as such they preserve brine strength by preventing fresh-water input and dilution. In addition, Zampirro suggests the NW-SE Cross Central Fault acts as a barrier to north-south flow across
the playa, as inferred by lithium mapping.
The central Clayton Valley is occupied by a flat lying playa that is 16 km long x 5 km wide, and covers an area of ~60 km2, representing an internal draining catchment of 1300 km2. The playa floor is at an altitude of 300 m asl, lower than both the neighbouring Big Smoky Valley to the NW and the Alkali Spring Valley to the NE, that are separated by low ridges. The Weepah Hills to the north have a maximum elevation of 2591 m, whilst the Silver Peak Range that limits the basin to the SW and west has maximum elevations of >2740 m. An elevated band of alluvium separates the Clayton and Big Smoky Valleys to the NW. The flat lying playa and the various ridges are separated by relatively gentle piedmont slopes composed of alluvium, which extend from the steeper slopes of the bordering ranges, spreading onto the playa to varying degrees. These boundary alluvial sediments tend to only contain fresh water and are not considered a lithium bearing unit of the mineral deposit (Davis et al., 1986).
Deposition within the Clayton Valley Basin is related to extended periods of relatively high and low precipitation (i.e., pluvial and inter-pluvial). The wetter periods produced fine-grained sediments, mainly muds in the valley centre in a lake environment, grading outward to peripheral fluvial and deltaic sands and muds, and then to beach sands and gravels on the valley margins. Lower energy deposits dominated in the drier periods, with deposition of muds, silt, sand and evaporites in the centre of the basin, with a relatively sharp transition to higher energy sand and gravel alluvial deposits on the boundary.
Throughout the Quaternary, the northeastern arm of the playa is interpreted to have been the primary location of subsidence and deposition, whilst the occurrence of thick evaporite and mud layers are taken to be indicative of the lake drying up during the low rainfall periods (Davis and Vine, 1979). The same authors also note the lake in Clayton Valley was likely shallow, relative to lakes in other contemporaneous Great Basin valleys, which are estimated to be as much as 200 to >1000 m deep. The presence of tuff and ash interbeds within the basin fill suggests atmospheric transport and deposition associated with large scale volcanic eruptions, most likely air fall and re-worked ash deposits from the Long Valley caldera, ~150 km to the NW, the main eruption of which was at ~0.76 Ma. The ash beds of the Lower Aquifer System (LAS) represent re-sedimented ash-fall associated with multiple, older volcanic events (Davis and Vine, 1979). Continued basin expansion during and after deposition resulted in normal faulting throughout the playa sedimentary sequence.
The stratigraphic succession that incorporates the six lithium-bearing aquifer systems and interbedded aquitards are as follows, from the base upwards:
Basement - composed of the ~2500 m thick late Neoproterozoic Reed Dolomite, conformably overlain by ~1000 m of the Late Neoproterozoic to Cambrian fine quartzites and siltstones of the Campito Formation, followed by 250 to 580 m of Poleta Formation limestones, and a further thickness of undifferentiated Palaeozoic metasedimentary siltstone. These are cut by Jurassic to Tertiary granitic rocks.
Erosional unconformity
Esmeralda Formation - sandstone, shale, marl, breccia and conglomerate, intercalated with volcanic rocks, deposited in several discrete Miocene basins (Davis et al., 1986). The age of the lower part of the Esmeralda Formation in the Clayton Valley is not known, but an air-fall tuff in the uppermost unit of the Formation has a K-Ar age of 6.9 ±0.3 Ma in the Late Miocene (Robinson et al., 1968).
Erosional unconformity - separating the Esmeralda Formation from the overlying Pleistocene sequence that hosts the 6 main aquifers. The thickness and lateral extent of these aquifers varies significantly, both across and along the length of the valley:
Lower Gravel Aquifer - LGA - which is the deepest aquifer and comprises gravel with a sand and silt matrix interlayered with clean gravel. It is considered alluvial material formed from the progradation of alluvial fans into the basin. Gravel clasts include limestone, dolomite, marble, pumice, siltstone and sandstone. Zampirro (2004) reports thicknesses of from 7.5 to >100 m. Ten wells drilled in 2021 and 2022 reached the base of the LGA, encountering thicknesses of from ~30 to ~190 m.
Erosional unconformity
Lower Aquifer System - LAS - predominantly composed of air-fall and reworked ash, likely from multiple volcanic sources (Davis and Vine, 1979). Individual ash beds are variably continuous, occurring as either lenses to discontinuous beds, or as extensive horizons. Zampirro (2004) reports that this unit extends from 100 to 300 m below ground level, and is interpreted to be moderately continuous north of the Cross Central Fault. Some of the thinner lenses are inferred to represent pluvial events carrying reworked ash possibly from surrounding highland areas into the lake environment. Lateral permeability in the LAS is limited where it occurs as narrow lenses of ash with lesser continuity.
Lower Lacustrine Facies - a thin aquitard composed of fine playa lake sediments.
Main Ash Aquifer - MAA - comprising air-fall and reworked ash, with particles ranging from submicroscopic to several centimetres or more as ash to pumice. The presumed source is from the eruption of the 0.76 Ma Long Valley caldera 40 km to the SW, which produced the Bishop Tuff. The latter is a welded tuff which formed as a rhyolitic pyroclastic flow. The MAA varies from 1.5 to 10 m in thickness and occurs from a depth of 60 m in the SW to >230 m in the NE. It is used as marker bed because of its continuity throughout the northeastern part of the valley.
Intermediate Lacustrine Facies - which includes fine playa sediments that act as an aquitard, and is comparable in thickness to the MAA and MGA that are below and above it respectively.
Marginal Gravel Aquifer - MGA - composed of silt, sand and gravel, and interpreted to be an alluvial fan deposits along the ENE Angel and Paymaster faults, where the majority of the basin down-faulting has occurred. Gravels are presumed to have been eroded from the basement on the uplifted footwall block of these faults (Zampirro, 2004). These faults are also interpreted to have acted as hydraulic barriers between the brines and freshwater.
Intermediate Lacustrine Facies - a thicker aquitard of fine playa sediments.
Salt Aquifer System - SAS - which is found in the northeastern segment of the valley, within its lowest section. It was formed by deposition in an arid lake, with precipitation of evaporites, primarily halite, from ponded water. It Includes lenses of salts, from <1 cm to 20 m in thickness, with interbeds of clay, some silt and sand, and minor gypsum, ash and organic matter. Some dissolution caverns are evident, having developed into sinkholes when pumped. Salt is inferred to have been precipitated in lowland standing water by concentration of minerals through evaporation. Deeper salt beds are more compact.
Upper Lacustrine Facies - another thicker aquitard composed of fine playa sediments.
Tufa Aquifer System - TAS - which lies in the northwestern segment of the valley. It is composed of travertine deposits, likely from either i). subaqueous vents that discharged fluid into the ancient lake, or ii). surficial hot spring terraces composed of CaCO3.
Uppermost Lacustrine Facies - another thick aquitard.
Alluvial Fan and Piedmont Deposits on the margin of the basin, thinning outward onto the bounding ridges.
Loess to Aeolian Deposits occurring as a thin veneer over the valley floor.
Mineralisation
The lithium resource at Silver Peak is hosted as a solute in a predominantly sodium chloride brine. Davis et al. (1986) suggest that the current levels of lithium dissolved in brine originate from relatively recent dissolution of halite by meteoric waters that penetrated the playa in the last 10 000 years. They suggest that the halite formed in the playa during the periods of low precipitation and that the concentrated lithium was incorporated as liquid inclusions into the halite crystals. Zampirro (2004) implies the lithium-rich rhyolitic tuff on the eastern margin of the basin were a possible source of the lithium in brine, as previously suggested by Kunasz (1970) and Price et al. (2000). Zampirro (2004) also notes the potential role of geothermal waters, either in leaching lithium from the tuff, or transporting lithium from the deep-seated magma chamber that was the source of the tuff.
Munk et al. (2011), by evaluating isotopic analyses of water and brine samples from throughout Clayton Valley, identified a complex array of processes affecting brine composition, that varied across a range of different locations. For brine in the Shallow Ash System (SAS), they identified a process that was consistent with that suggested above by Davis et al. (1986). Their results support a process whereby lithium was co-concentrated with chloride and then trapped in precipitated sodium chloride (halite) crystals. However, in brine samples from other locations they found evidence that lithium was not co-concentrate with chloride, but was introduced to the brine by fluids that already contained elevated lithium levels. Their results were consistent with lithium leached from the lithium-bearing clay mineral hectorite, being accumulated within the basin via two possible mechanisms. The first mechanism involves water interacting with hectorite to the east of the basin, and subsequent transport into the basin. The second involves leaching of hectorite within the basin sequence, where it had formed through alteration of volcanic sediments.
According to Davis et al. (1986), there is no evidence of anomalous amounts of lithium in pre-Tertiary rocks. The Tertiary volcanic rocks, however, may have provided the heat energy and hydrothermal fluids required to mobilise lithium from volcanic glass and other relatively unstable minerals. About 100 km3 of lava erupted from the Silver Peak volcanic center in the western part of Clayton Valley ~6 m.y. ago (Robinson, 1972). To the east, another 100 km3 of Tertiary ash-flow and air-fall tuff is exposed at Clayton Ridge and as far east as Montezuma Peak. These predominantly flat-lying, pumiceous rocks are interbedded with tuffaceous sediments between Clayton Ridge and Montezuma Peak. At Montezuma Peak these rocks are altered considerably, and in the Montezuma Range, they are unconformably overlain by rhyolitic agglomerates. Hydrothermal alteration of these voluminous volcanic rocks in the immediate catchment of Clayton Valley may have been the ultimate source of lithium. Extensive diagenetic alteration of vitric material has been observed, and high lithium concentrations are associated with smectite alteration in the Miocene Esmeralda Formation exposed east of Clayton Valley (Davis et al., 1986).
Resources and Reserves
Mineral Resources as of 30 September, 2022 (SRK Consulting Technical Report Prepared for Albemarle Corporation, 2023):
Measured Resource - 14 000 tonnes of contained Li metal in a brine containing 153 mg/L Li;
Indicated Resource - 36 200 tonnes of contained Li metal in a brine containing 144 mg/L Li;
Inferred Resource - 89 500 tonnes of contained Li metal in a brine containing 121 mg/L Li.
TOTAL Resource - 139 700 tonnes of contained Li metal in a brine containing 130 mg/L Li.
Ore Reserves as of 30 September, 2022 (SRK Consulting Technical Report Prepared for Albemarle Corporation, 2023):
Proved Reserve (in situ) - 12 000 tonnes of contained Li metal in a brine containing 94 mg/L Li;
Probable Reserve (in situ) - 56 300 tonnes of contained Li metal in a brine containing 95 mg/L Li;
Proved + Probable Reserve (in situ) - 68 300 tonnes of contained Li metal in a brine containing 95 mg/L Li.
NOTE: Resources are exclusive of Reserves. Li2CO3 contains 18.78% Li metal.
Process recovery is estimated based on historical operational performance through a combination of a fixed 78% recovery rate in the lithium carbonate plant and a variable pond recovery factor, based on raw brine lithium concentration that averages around 51% over the reserve life.
The nameplate capacity of the lithium carbonate plant is listed as 6000 t/y Li2CO3. However, in recent years Silver Peak has demonstrated that the plant is capable of producing higher than that. In 2018, the plant produced approximately 6500 tonnes of Li2CO3.
The information within this summary has been largely drawn from SRK Consulting (U.S.), Inc., 2023 - SEC Technical Report Summary, Pre-Feasibility Study, Silver Peak Lithium Operation, Nevada, USA; prepared for Albemarle Corporation, Charlotte, North Carolina, 215p.
The most recent source geological information used to prepare this decription was dated: 2023.
This description is a summary from published sources, the chief of which are listed below. © Copyright Porter GeoConsultancy Pty Ltd. Unauthorised copying, reproduction, storage or dissemination prohibited.
Silver Peak Lithium
|
|
|
Davis, J.R. and Vine, J.D., 1979 - Stratigraphic and Tectonic Setting of the Lithium Brine Field, Clayton Valley, Nevada.: in Rocky Mountain Association of Geologists - Basin and Range Symposium, Proceedings, pp. 431-430.
|
Davis, J.R., Friedman, L. and Gleason, J.D., 1986 - Origin of lithium-rich brine, Clayton Valley, Nevada: in U.S. Geological Survey Bulletin B1622, pp. 131-138.
|
Zampirro, D., 2004 - Hydrogeology of Clayton Valley Brine Deposits, Esmeralda County, Nevada: in Nevada Bureau of Mines and Geology Special Publication 33, pp. 271-280.
|
Porter GeoConsultancy Pty Ltd (PorterGeo) provides access to this database at no charge. It is largely based on scientific papers and reports in the public domain, and was current when the sources consulted were published. While PorterGeo endeavour to ensure the information was accurate at the time of compilation and subsequent updating, PorterGeo, its employees and servants: i). do not warrant, or make any representation regarding the use, or results of the use of the information contained herein as to its correctness, accuracy, currency, or otherwise; and ii). expressly disclaim all liability or responsibility to any person using the information or conclusions contained herein.
|
Top | Search Again | PGC Home | Terms & Conditions
|
|