Olympic Dam, OD Deeps |
|
South Australia, SA, Australia |
Main commodities:
Cu U Au
|
|
 |
|
 |
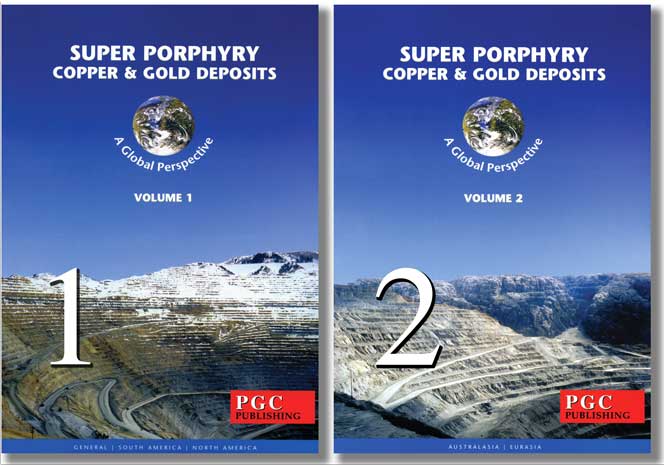 |
Super Porphyry Cu and Au

|
IOCG Deposits - 70 papers
|
All papers now Open Access.
Available as Full Text for direct download or on request. |
|
 |
The Olympic Dam copper-gold-silver-uranium-REE ore deposit is located ~560 km NNW of Adelaide and 275 km NNW of Port Augusta, in northern South Australia (#Location: 30° 26' 24"S, 136° 53' 22"E).
Upate March 2023 - In 2020-21, a review of the mineralisation potential at depth resulted in an exploration drilling program that in 2022-23 outlined the major structurally controlled OD Deeps zone beneath the main resource envelope. That zone is described separately below, following the 'structure' section.
Go to: Regional Setting, Geology, Structure, OD Deeps Zone, Alteration, Mineralisation, Geophysical Expression, Evolution and Discussion and Reserves and Resources.
DISCOVERY and HISTORY
The Olympic Dam deposit was discovered in July 1975 by Western Mining Corporation Ltd (WMC; Reeve, 1990) and was the first deposit of its type recognised, leading to the creation of the iron oxide copper-gold (IOCG) deposit model by Hitzman, Oreskes and Einaudi (1992). The discovery was the result of exploration for sediment hosted copper mineralisation, based on a conceptual model advanced by Haynes in 1972 (Haynes, 2006). This model envisaged alteration of continental tholeiitic basalt to an assemblage of albite-hematite-phyllosilicates-epidote-carbonates by deeply circulating oxidised fluids. It was postulated that this alteration process would have released copper, mainly hosted in magnetite within the volcanic rocks, to be taken into solution by these fluids. It was suggested these copper-pregnant fluids then migrated up steeply dipping faults until encountering reduced sedimentary rocks where copper was precipitated in a sedimentary trap, such as a domal structure (Haynes, 1972).
The ~1590 Ma Roopena Volcanics in South Australia were identified as a potential example of copper-depleted altered continental tholeiitic basalts. These basalts underlie the flat-lying Meso- to Neoproterozoic to Lower Palaeozoic sedimentary sequence of the Stuart Shelf. Prospective targets were delineated by geophysical data in conjunction with lineament analysis (Rutter and Esdale, 1985; O'Driscoll, 1985; Esdale et al., 2003). Coincident gravity and magnetic anomalies were considered to reflect the geophysical expression of mafic volcanic rocks in the core of a domal structure, or in a fault bounded basement high, that might satisfy the proposed model. The first drill hole at Olympic Dam was sited on such a geophysical target, developed at the intersection of WNW and NNW lineaments. The target was was developed from geophysical data from pre-competitive surveys by the Commonwealth Bureau of Mineral Resources and the South Australian Department of Mines and Energy.
The discovery diamond hole, RD1, drilled over this anomaly in July 1975, intersected 38 m @ 1.05% Cu, 70 ppm U3O8, 0.27 g/t Au, 3.5 g/t Ag at a depth of 353 m beneath the surface (Roberts and Hudson, 1983). There was an apocryphal rumour circulating in the early 1980s that the mineralisation was not visually obvious, and the interval was only assayed after the core was left in the open for some months and the fine sulphides had begun to oxidise. The next eight drill holes contained very low to low-grade copper. High-grade mineralisation was not intersected again until 15 months after RD1, in drill hole RD10, which intersected 170 m @ 2.09% Cu, 630 kg/t U3O8, 0.65 g/t Au, 3.1 g/t Ag). RD3, 4, 6 and 7 had been barren, RD5 and 8 intersected additional mineralisation containing ~1% Cu, while RD9 was lower grade. The RD10 intersection was the first indication of potentially economic grades of both copper and uranium, and provided the impetus for the initiation of a comprehensive surface drilling program that resulted in an estimate of >2 Gt @ 1.6% Cu, 0.06% U3O8 and 0.6 g/t Au. Drilling over an area of 25 km2 resulted in a success rate of >80% mineralised drill holes (Roberts and Hudson, 1983). That drill program was undertaken under a joint venture signed in mid-1979 between WMC (51 %) and the British Petroleum Group (49%).
The host sequence was interpreted to be basement to the Neoproterozoic and younger sedimentary rocks of the Stuart Shelf, and to predominantly comprise a >1 km thick succession of sedimentary breccias that ranged from matrix-poor with granite clasts, to matrix-rich polymict breccias containing clasts of a variety of rock types. These sedimentary breccias were interpreted to have been deposited within a NW-SE elongated graben. The sequence was divided into, i). the Olympic Dam Formation, with five members, three of which are matrix rich; and the overlying ii). Greenfield Formation, with three members, the lower two being very hematite rich while the upper has a significant volcanic component. Pervasive hematite, chlorite and sericite alteration of varying intensity was interpreted to have affected all the members of both formations. The basement below the Olympic Dam Formation was assumed to be a granite, equivalent to that found outside of the known area of mineralisation (Roberts and Hudson, 1983).
Mineralisation was interpreted to comprise stratabound bornitic (chalcocite)-chalcopyrite-pyrite in the hematite matrix-rich members of the Olympic Dam Formation, with sulphides mainly occurring as uniform disseminations in the breccia matrix, although up to 5 cm clasts of massive sulphides were also observed. A vertical zonation of sulphides was noted, ranging from a sulphur-rich, copper-poor pyrite (-chalcopyrite) at the base of the Olympic Dam Formation, to a copper-rich and sulphur-poor assemblage of bornite (-chalcocite) at the top. No evidence of supergene enrichment or lateral zoning of sulphides was noted. However, a distinct siderite-rich zone was seen to follow the northeastern margin of the deposit, in which pyrite is the dominant sulphide. Intense chlorite alteration was interpreted to have developed at the base of the stratabound mineralisation, whilst transgressive chalcocite-bornite was observed to overprinted the stratabound mineralisation forming a 6 by up to 0.7 km NW-trending linear corridor in the centre of the proposed graben. Massive sulphide clasts, veins, clast rims and cavity infill were observed, complexly intergrown with the chalcocite-bornite. Sericite-quartz or chlorite alteration halos were observed, developed around sulphide veins. In both the stratabound and transgressive mineralisation, hematite, quartz, sericite and fluorite were observed to be the principal gangue minerals, with lesser chlorite, siderite, barite and rutile. Very fine grained uraninite, with lesser coffinite and brannerite were noted to occur in a variety of forms, whilst the rare earth minerals bastnaesite and florencite were found in and adjacent to, sulphide mineralised zones. The interpretations of Roberts and Hudson (1983) were based on relatively sparse surface drilling alone.
An exploration shaft was sunk to a depth of 500 m in 1982, and underground development through the mineralised zones commenced. Detailed underground
drilling and geological evaluation followed, during which it was realised that the Olympic Dam mineralisation was not hosted by a sedimentary breccia, but was associated with intense, texturally destructive hematite and sericite alteration and brecciation of the primary host rock units, including the ~1593 Ma Roxby Downs Granite, 'bedded clastic facies' rocks, and mafic-ultramafic dykes, lavas and sills. This realisation led to a reappraisal of the deposit's origin, the most accepted of which involved a maar-diatreme setting, where fluid mixing was responsible for alteration and metal precipitation in a near-surface regime, temporally and spatially associated with the extensive ~1590 Ma Gawler Range Volcanics - Hiltaba Suite event (Reeve et al., 1990; Haynes et al., 1995; Johnson and Cross, 1995). In this model, the Olympic Dam and Greenfield formations of Roberts and Hudson (1983), broadly coincided with the altered and mineralised Roxby Downs Granite and maar crater sediments respectively. A less widely accepted model, proffered by Oreskes and Einaudi (1990, 1992), involved a faulted basin and multiple fluid sources, where the hydrothermal system and brecciation was activated ~100 m.y. after the Gawler Range Volcanics - Hiltaba event, and subsequently modified by supergene processes. Following a further major ~450 km drilling program of close spaced holes, McPhie et al. (2011, 2016) showed that the volcaniclastic and minor sedimentary units previously interpreted to have been deposited in maar craters, were a series of thick, laterally continuous 'bedded clastic facies' largely deposited below wave base in a subaqueous sedimentary basin. These volcano-sedimentary rocks have been faulted and incorporated into the breccia complex, where brecciation that was initiated along faults allowed the deep circulation of fluids. Explosive injection of multiple mafic and ultramafic dykes in a wet environment, resulted in further local brecciation (McPhie et al., 2011).
In April 1993, WMC (Olympic Dam Corporation) Pty Ltd purchased the BP Group 49% share. Then, in June 2005, the BHP Billiton Group acquired WMC Resources Ltd., and the Olympic Dam operation was merged into the former, which in November 2018 became BHP Group Limited.
REGIONAL SETTING
Olympic Dam, and the other significant known IOCG mineralised systems of the Mesoarchaean to Mesoproterozoic Gawler Craton, are hosted within Palaeo- to Mesoproterozoic rocks, and are distributed along the eastern rim of the currently preserved craton to define the Olympic IOCG Province (Skirrow et al., 2007). Olympic Dam lies below the Neoproterozoic Stuart Shelf, where >300 m of flat lying, largely barren, Neoproterozoic to lower Palaeozoic sedimentary rocks unconformably overlie both the craton and the ore deposit. However, regionally, sections of this cover sequence do contain sediment hosted Cu mineralisation, some of which has been mined (e.g. Mount Gunson, Cattle Grid). Some 75 km to the east, this cover sequence expands across the major NNW trending Torrens Hinge Zone at the edge of the craton, into the thick succession of the north-south aligned Neoproterozoic Adelaide Geosyncline rift basin. This rift basin masks the mid- to late-Palaeoproterozoic suture between the Gawler craton and Palaeo- to Mesoproterozoic Curnamona Province to the east.
For a more detailed description of the tectonic and geologic setting of the Olympic IOCG Province and the Gawler Craton, see the separate Gawler Craton and Olympic IOCG Province record.
In summary, the oldest rocks in the Gawler Craton include the ~3.40 to 3.15 Ga Cooyerdoo Granite and underlying Palaeoarchaean TTG basement, followed by further felsic magmatism represented by the ~2820 Ma Coolanie Gneiss. These units form the core of the craton, but are only exposed as windows through younger sequences on the northeastern Eyre Peninsular to the west of the Olympic IOCG Province. Extensive Neoarchaean complexes deformed by early Palaeoproterozoic orogenesis comprise the gneissic Mulgathing and Sleaford complexes in the northern and central-southern sections of the craton. The southern and eastern Gawler Craton, within and to the immediate west of the Olympic IOCG Province, is underlain by the 1.96 to 1.85 Ga Hutchison Group volcano-sedimentary rocks, 1855 to 1845 Ma Donington Suite granitoids and the 1.76 to 1.74 Ga volcano-sedimentary Wallaroo Group and equivalents. The major 2.48 to 2.42 Ga Sleafordian and 1.73 to 1.69 Ga Kimban orogenies/metamorphic events affected the Archaean and Palaeoproterozoic units respectively (Hand, Reid and Jagodzinski, 2007).
The eastern Gawler Craton contains a major lithospheric boundary, interpreted to be a palaeo-suture (Hayward and Skirrow, 2010; Betts et al., 2015). The precise location of this boundary is uncertain in the Olympic Dam area. Betts et al. (2015) suggest this suture corresponds to the NW-SE Elizabeth Creek Fault that forms the western margin of the Olympic IOCG Province. However, a major linear discontinuity in magnetic and gravity data beneath the Neoproterozoic Adelaide Geosyncline intracratonic rift complex to the east is also suggested to represent this structure (Hayward and Skirrow, 2010, and sources quoted therein), an option that is more likely. Betts et al. (2015) argue that the suture initially formed at ~1850 Ma, during the Cornian Orogeny, when the Gawler Craton was accreted to the North Australian Craton, a remnant of which is the Curnamona Block. This orogeny is reflected by the emplacement of the Donington Suite intrusives which were extensively developed along the northern Olympic IOCG Province. Betts et al. (2015) further suggest that the South Australian/Gawler and North Australian Craton were subsequently rifted apart during a period of extension and basin development (including deposition of the Wallaroo Group) that lasted until ~1740 Ma, and that the two terranes were finally re-sutured during the 1730 to 1690 Ma Kimban Orogeny.
The Mesoproterozoic succession is dominated by the ~1595 to 1545 Ma Hiltaba/Gawler Range silicic large igneous province (Jagodzinski et al., 2021), which is mainly composed of the largely undeformed, subaerial, Gawler Range Volcanics (GRV; Giles, 1988; Blissett et al., 1993; Allen et al., 2003, 2008).The GRV is estimated to have totalled ~90 000 km3 of magma (McPhie et al., 2008). These volcanics, although bimodal, are largely felsic with lesser mafic varieties and were predominantly deposited between 1595 and 1575 Ma in two suites, a Lower, ~1592 Ma, and Upper, ~1588 Ma Gawler Range Volcanics. They effectively bracket the extensive A- and I-type felsic granitoids and lesser mafic rocks, including gabbros, of the 1598 to 1583 Ma Hiltaba Suite (Fanning et al., 1988; Flint, 1993), with the former dominating in the Olympic IOCG Province. The Lower GRV includes dacite to rhyolite, ignimbrites and tuffs with minor to locally significant basalt erupted from discrete volcanic centres. Basaltic to andesitic rocks are most prevalent along the western margin of the Olympic IOCG Province, from ~50 km SE of Prominent Hill to ~100 km south of Carrapateena. The upper GRV comprises three regionally extensive, 200 to 300 m thick, high temperature (900 to 1100°C) felsic lava flows. The various volcanic centres of the Lower GRV are considered to be derived from fractional crystallisation and crustal contamination of mantle melts (Fricke 2005; Creaser 1995; Stewart 1994; Giles 1988), whereas the flows of the Upper GRV are isotopically homogenous and define a single fractionation trend, suggesting that they were derived from the periodic tapping of a single stratified magma reservoir (McAvaney and Wade 2015; Stewart 1994).
Through Pb-isotopic analyses of alkali feldspar in the Hiltaba Suite and GRV, Chapman et al. (2019) revealed a craton-wide isotopic zoning. Pb-isotopic zonation is controlled by the varying interaction of a parental melt derived from i). a lithospheric mantle-like enriched reservoir which exerts a strong control on Pb-isotopic compositions around the central-western Gawler Craton (Nuyts Terrane); and two crustal end-member reservoirs which are ii). an unradiogenic reservoir which represents granulitic lower crust, and appears to be spatially limited to the northwest of the Gawler Craton; and iii). a radiogenic reservoir, which is more prevalent in the eastern Gawler Craton, coincident with the IOCG province and probably represented by monazite-rich metasediments.
The Hiltaba Suite and Gawler Range Volcanics are oldest in the immediate Olympic Dam area, with a weighted average age of 1592.62 ±0.66 Ma (Jagodzinski et al., 2021). Most of the magmatism dated has returned ages of between ~1595 and 1575 Ma, with ~14% younger, between ~1575 and 1545 Ma. The latter are concentrated around the margin of the Gawler Craton in the Mount Painter and Mount Babbage Inliers, as well as on Yorke Peninsula, Peake and Denison Ranges and Fowler Domain, and in the Curnamona Province (Jagodzinski et al., 2021).
McPhie et al. (2011) showed via analyses of melt inclusions in quartz phenocrysts from rhyolite of the Gawler Range Volcanics, that the silicic magmas of this large igneous province were unusually rich in F, containing up to 1.3 wt.% F. As a consequence they infer any associated fluid would contain abundant hydrofluoric acid, the most corrosive acid known, accelerating any fluid-rock and fluid-metal interaction.
These rocks were locally affected by the 1565 to 1540 Ma Kararan Orogeny that led to the reactivation of faults in the northern and western Gawler Craton (e.g., Ehrig et al., 2017).
Around Olympic Dam, in the eastern Gawler Craton, the rocks of the large igneous province are overlain by the continental-derived sedimentary rocks of Calymmian Pandurra Formation, deposited within the Cariewerloo Basin between 1575 and 1425 Ma associated with an inferred extensional regime that bracketed that period. In situ Pandurra Formation has not been formally recognised immediately below the Neoproterozoic unconformity at Olympic Dam. However, as detailed below, a narrow sliver of well-sorted quartz-rich sandstone containing detrital zircon grains with an age spectrum identical to that the Pandurra Formation is structurally interleaved with the host sequence within the Olympic Dam mine (Ehrig et al., 2017). It is also possible that the 'bedded clastic facies' within the Olympic Dan Breccia Complex are the precursors of the Pandurra Formation. The Pandurra and older formations were intruded by the 180 to 250 km wide, NE-SW trending, ~825 Ma, mafic-ultramafic Gairdner Dyke Swarm (Zhao and McCulloch, 1993; Zhao, McCulloch and Korsch, 1994), and unconformably overlain by the, extensive, mainly flat lying, Neoproterozoic to Cambrian sedimentary rocks of the Stuart Shelf (Preiss, 1993).
GEOLOGY
The Olympic Dam iron oxide Cu-U-Au-Ag deposit is truncated by a major unconformity at the top of the Mesoproterozoic basement, below the cover sequence described above. The delineated resource covers an area of ~6 x 3 km and is relatively thin with a vertical extent that generally extends no more than 800 m below that basement surface, except in the southeastern segment where it has been tilted and down-faulted to depths of as much as 2 km or more below the unconformity.
The iron oxide Cu-U-Au-Ag mineralisation of the deposit is hosted entirely within the Olympic Dam Breccia Complex that is principally developed within the ~1593 Ma Roxby Downs Granite of the regional Hiltaba Suite (Reeve et al., 1990). Other less abundant rock units within the Olympic Dam Breccia Complex include felsic and mafic to ultramafic volcanic rocks of the marginally older, but comagmatic Gawler Range Volcanics, as well as a thick succession of overlying 'bedded clastic facies' rocks that are marginally younger than the Roxby Downs Granite (McPhie et al., 2011, 2016). The Roxby Downs Granite intrudes the Palaeoproterozoic Wallaroo and Hutchison groups that comprise meta-sedimentary and meta-volcanic rocks and iron formations, as well as variably deformed Donington Suite megacrystic granite, and ~1590 Ma Gawler Range bimodal ultramafic to felsic lava and sills, and ignimbrites. All of these rocks are unconformably overlain by an ~350 m thick cover sequence of the Stuart Shelf that comprises flat-lying, undeformed, post-brecciation and post-mineralisation Neoproterozoic to Cambrian sedimentary units. The Neoproterozoic Gairdner Dyke Swarm dolerite and basalt intrude all basement lithologies at Olympic Dam (Reeve et al., 1990; Ehrig, McPhie and Kamenetsky, 2012; Huang et al., 2015).
Ehrig (2019) emphasised that during and over the succeeding billion years after its deposition, the Olympic Dam deposit has been substantially structurally dislocated, such that the present orebody geometry does not reflect the pre- or syn-mineralisation structural architecture, but rather reorganised and partially remobilised remnants. During this same extended period, the deposit has been influenced and modified by a number of tectonic events and pulses of hydrothermal fluid circulation, including, in particular, upgrading by addition of further uranium between 0.7 and 0.5 Ga (Ehrig et al., 2021).
Click here to expand -or- here for a location map
Click here for cross section location map
The geology within the deposit area has been subdivided as follows, after Ehrig et al. (2012, 2017) and McPhie et al. (2016):
NOTE: Sericite and muscovite are used interchangeably in this and source descriptions, and include sericite, fine-grained muscovite, illite, phengite and paragonite.
Pre-brecciation lithologies
RDG - Roxby Downs Granite, which is principally an undeformed, pink to red, medium- to coarse-grained (<2 cm), hypidiomorphic, equigranular to slightly porphyritic granite that contains ~50 wt.% alkali feldspar, 21 to 25% quartz, 17 to 23% sodic plagioclase and 5 to 7% biotite and amphibole, accompanied by accessory to trace igneous magnetite, titanite, apatite, zircon, allanite, fluorite, tourmaline, calcite-ankerite, synchysite, uranothorite and trace sulphides (Ehrig, McPhie and Kamenetsky, 2012). Alkali feldspars (~Or55 Ab43 An2) which are coarsely perthitic, and plagioclase with two compositions (~An12-20 and ~An27-34)
display both rapakivi and anti-rapakivi, and occasionally pseudogranophyric textures (Kontonikas-Charos et al., 2017). Unaltered Roxby Downs Granite is characterised by the presence of biotite, K2O/Na2O ratios of 5.5:3.5, magnetic susceptibility range of ~25 to 50 x 10-3 SI units, and a bulk dry density of ~2.65 to 2.70 g/cm3. The Roxby Downs Granite has been dated at 1593.3 ±1.2 Ma (SHRlMP, U-Pb zircon; Jagodzinski, 2014) and 1593.87 ±0.21 Ma (Cherry et al., 2018). The age and contact relationships of the Roxby Downs Granite suggest it intruded the overlying, broadly comagmatic Gawler Range Volcanics which have been dated at 1594.73 ±0.30 Ma; (Cherry et al., 2018).
Felsic Volcanic Units - Although no in situ felsic lavas have been mapped at Olympic Dam, remnants of feldspar-phyric felsic lavas occur as angular porphyritic fragments in hematite breccias and as clasts in some of the 'bedded clastic facies' (McPhie et al., 2011, 2016; Ehrig, McPhie and Kamenetsky, 2012). Zircons from these felsic fragments have been dated at ~1590 Ma (Johnson and Cross, 1995) and are interpreted to have been derived from the regionally extensive Gawler Range Volcanics (Ehrig et al., 2017).
Pre- to Syn-brecciation Units
Bedded Clastic Facies, as preserved, occur as an up to 350 m thick packages within, but mainly near the top of, the Olympic Dam Breccia Complex, immediately below the Neoproterozoic unconformity, although large blocks have been down-faulted to deeper levels. Contacts between these facies and the hematite-rich breccias are commonly faulted, but are elsewhere gradational. Determination of the relationship is complicated by the intense alteration that has overprinted the contacts. However, at gradational contacts, the 'bedded clastic facies' are commonly brecciated and separated from hematite-rich or granite-rich breccias by intervals composed of fragments of the 'bedded clastic facies' dispersed through hematite-rich or granite-rich breccia, whilst domains of hematite-rich breccia also cut across the bedded sedimentary facies (McPhie et al., 2011). Never-the-less, the 'bedded clastic facies' rocks are markedly different to the hematite-rich breccia, distinguished by their overall finer grain size and well-defined planar bedding.
McPhie et al. (2011) note that these facies are composed of laminated to very thinly bedded mudstone, thinly to medium-bedded sandstone, and thickly bedded conglomerate. They include both red, hematite-rich, and green, chlorite-rich mudstone and sandstone intervals that can be tens of metres thick. The red, hematite-rich sandstone intervals include abundant granite fragments and granite-derived feldspar and quartz, whilst those in the green, chlorite-rich intervals are mainly composed of mafic volcanic fragments, as well as containing detrital chromite. Whilst the mafic volcanic fragments match those of the Gawler Range Volcanics, the chromite grains do not, and appear to have been derived from separate sources and mixed by surface sedimentary processes prior to final deposition. Volcanic quartz (<5 modal %) is found in most of both red and green sandstone, although while such quartz-bearing volcanic units occur in the Gawler Range Volcanics, none are exposed proximal to Olympic Dam. The conglomerate beds comprise well-rounded, moderately sorted, feldspar-phyric felsic and mafic volcanic clasts and minor granite fragments (McPhie et al., 2011). These facies, which have been variably modified by hydrothermal activity, are interpreted to be mainly sourced from the Gawler Range felsic and mafic volcanics and Hiltaba Suite felsic intrusions, although Archaean and Palaeoproterozoic zircons in well-sorted quartz-rich sandstone indicate that distal older Gawler Craton basement also contributed detritus (McPhie et al., 2011).
Accumulation of the 'bedded clastic facies' was at least partly contemporaneous with explosive rhyolitic eruptions from vents that were most likely tens of kilometres or more distal, indicated by bubble-wall shards found in tuffaceous mudstone facies (McPhie et al., 2011). As such the sequence overlaps the waning stages of the Gawler Range Volcanics. The laminated mud and graded sand beds are typical of below-wave-base subaqueous settings, whilst the conglomerate beds are likely to be deposits from high-concentration gravity currents such as debris and turbidity flows. The preserved sections of these rocks suggest that the un-compacted sedimentary thickness was >500 m. The area covered by the sedimentary basin is unknown, although the dominance of subaqueous below-wave-base facies, its substantial thickness, and presence of exotic detritus imply it was much more extensive than the preserved bedded sedimentary facies at Olympic Dam, and is not consistent with deposition confined to a maar crater, either with regard to area, thickness or facies of the sedimentary sequence. The extent and character of this basin are interpreted to imply the the Olympic Dam hydrothermal system operated beneath, and partly within, the basal sequences of an active sedimentary basin, and did not significantly vent to surface (McPhie et al., 2011; 2016).
The 'bedded clastic facies' were deformed prior to final lithification, attested to by folds that have diverse shapes and sizes but lack cleavage. These folds have a gentle and reasonably consistent axial plunge to the NE and may be related to slumping on a NE-striking palaeoslope, dipping either to the NW or SE. The depocenter in which they were accumulated is interpreted to have been bounded by a combination of NE- and NW-striking faults (McPhie et al., 2016). Ehrig et al. (2021), after Cherry et al. (2018) show that the age of for the 'bedded clastic facies' tuffaceous mudstone has been constrained to 1590.97 ±0.58 Ma, which implies the probable detritus source, the Roxby Downs Granite, was partially exhumed before ~1591 Ma (Cherry et al., 2018). This is taken to indicate the presence of a basin by ~3 m.y. after emplacement of the granite and felsic volcanic rocks.
McPhie et al. (2016) consider it likely that the 'bedded clastic facies' were deposited in a fault bounded sedimentary basin while the Olympic Dam hydrothermal system was active. As such, that system was probably responsible for sub-sea floor brecciation and alteration within the underlying Roxby Downs Granite and Gawler Range Volcanics basement during deposition of the 'bedded clastic facies' and subsequently progressively stoped upwards to also intersect and breccite the latter. In this sense, the 'bedded clastic facies' are pre- to syn-brecciation.
The 'bedded clastic facies' comprise the following main associations, not necessarily in stratigraphic order:
• KASH - laminated, intercalated green sandstone and mudstone, which is up to 100 m thick and can be traced over a 1.3 km strike length and to a depth of ~750 below the Neoproterozoic unconformity. It comprises interlaminated green sandstone and mudstone, with ~1 m, and occasionally up to 10 m thick green sandstone intercalations, which contain green mudstone intra-clasts. The dominant green colouration of the mudstone is caused by phyllosilicates, mainly chlorite, although fine-grained sericite is also present (McPhie et al., 2016). The sandstone and mudstone generally have sharp contacts, although some sandstone beds grade into mudstone. Laminae and beds are laterally continuous and of even thickness. It is dominantly made up of chlorite-altered mafic volcanic and lesser sericite-altered feldspar-phyric felsic volcanic clasts, as well as chlorite-altered ferromagnesian and sericite-altered feldspar grains that include relict orthoclase and albite, with quartz, carbonate, fine-grained white mica and accessory zircon and titanite. This detritus was derived from mafic volcanic and granitoid rocks. The altered mafic igneous clasts have porphyritic, doleritic and vesicular textures, and a composition suggestive of chlorite-pseudomorphed olivine crystals that contain chromite inclusions and free chromite crystals (McPhie et al. , 2016). As such it is rich in detrital chromite, and is characterised by ~9 wt.% Al, 2.5% Mg, 3.5 to 4.5% K and 0.45 to 0.55% Ti, with elevated 400 to 500 ppm Cr, 100 to 200 ppm V, 100 to 300 ppm Ni and 200 to 400 ppm Zn (Ehrig et al, 2012). Most green sandstones and mudstones sampled also contain more abundant P2O5 (>0.2 wt.%) than the red sediments of the KHEMQ (McPhie et al. , 2016). The sandstone contains interstitial sulphide minerals, including pyrite, chalcopyrite, bornite and chalcocite, together with siderite, barite, fluorite and apatite, whilst minor sulphides are also present within cross-cutting barite ±carbonate veins. Variably brecciation, alteration and subordinate mineralisation is observed throughout the unit, and in addition to the chlorite and sericite, it has experienced hematite alteration where it assumes a reddish-brown colouration. Despite the alteration and presence of sulphides, the mean copper concentration of the unit is only 0.21 wt.% and is therefore largely barren (McPhie et al. , 2016).
However, the distribution of mineralisation is strongly influenced by KASH, whereby increasing copper grades are observed proximal to its faulted contact. A contiguous, albeit narrow zone of high-grade mineralisation occurs along the southernmost faulted contact of KASH, forming a local small extension to the main orebody. This segment of the unit has been significant structurally disturbed, characterised by intense hematite + sericite alteration, with textural destruction of sedimentary features. It is uncertain as to whether this zone is due to post-mineral ‘reworked’ brecciation and remobilisation of copper to oxidized, chalcocite-dominant ore, although it appears likely (Clark and Ehrig, 2019). The >1% Cu mineralisation shown within KASH on Fig. 2 is likely influenced by the proximity of high grade mineralisation near its contact.
• KHEMQ - well-bedded, intercalated, hematite-quartz-rich sandstone and red mudstone, with localised conglomerate-breccias. This unit comprises up to 50% of the 'bedded clastic facies', with preserved thicknesses of up to 90 m. It is composed of hematite, granite and felsic volcanic clasts, and granitoid- and volcanic-derived quartz and feldspar fragments, but lacks significant mafic igneous components. It is mainly composed of red mudstone, which is almost entirely composed of red and grey hematite, with minor magnetite and scattered fine quartz, zircon, white mica and Fe-chlorite. The red mudstone intervals are planar laminated to very thinly bedded. Some mudstone bands are delicately laminated and hematite-rich, with successive laminae of red, grey and black hematite that resemble banded iron formation. The KHEMQ facies association contains anomalous Mo and As of ~150 and >200 ppm respectively, which has been interpreted to represent a hydrothermal overprint. The red mudstone is intercalated with coarse- to fine-grained, thinly, 3 to 10 cm, bedded and laminated white, yellow and pink sandstone. Some thicker, up to 30 cm, sandstone beds and 50 cm thick polymictic, granule to pebble conglomerate and breccia beds are also present. The polymictic conglomerate and breccia beds generally display normal grading upwards into parallel laminated sandstone. Granitoid clasts and granitoid-derived quartz and feldspar are the dominant components of the sandstone, which also contains hematite, zircon, tourmaline, titanite and rutile. The thicker sandstone beds are typically internally massive, parallel laminated or graded, and tabular, although some are lenticular. These sandstones exhibit flame, and ball-and-pillow structures, cross-beds and cross-laminae, defined by dark bands of grey hematite. Quartz, fine-grained white mica and hematite are common sand grains, with interstitial sulphides that include pyrite, chalcopyrite, bornite and chalcocite, with local florencite and Pb telluride (McPhie et al. , 2016).
• VASH - thinly laminated, hematite-rich tuffaceous mudstone, which is a delicately interlaminated green and red, very fine grained (<1 mm), strongly hematite-rich, tuffaceous mudstone facies with minor laminae to thick beds of fine-grained, pale grey, pale brown and purple sandstone. The red mudstone layers are composed of fine-grained hematite and white mica, and the fine-grained sandstone beds contain quartz, feldspar and angular to subangular felsic volcanic clasts. Some of the sandstones are normally graded. Laminae and thicker beds are laterally continuous and of even thickness. Some laminae and thin beds of green mudstone contain sparse chromite grains, and others include magnetite rimmed by hematite. The felsic tuffaceous mudstone contains well preserved bubble-wall shards, fine angular volcanic quartz and zircon. This latter mudstone is pale green, and occurs in internally massive laminae and thin to thick beds (McPhie et al. , 2016).
• KFMU - polymictic conglomerate with felsic and mafic clasts of Gawler Range Volcanics. This unit is only a minor, but locally up to 70 m thick, component of the 'bedded clastic facies', and is in faulted contact with the VASH unit. The predominantly conglomerate facies is interbedded with structurally underlying green sandstone and mudstone and an overlying interbedded hematite-rich sandstone and red mudstone facies. It is distinguished by its much coarser grain size, with most clasts being 1 to 6 cm in diameter. The conglomerate is characterised by pink to red hematite- and green chlorite-altered volcanic cobbles and pebbles. Most clasts are feldspar-phyric and felsic in composition, and very similar to feldspar-phyric rhyolites of the Gawler Range Volcanics. Less than 1% of the felsic volcanic clasts are quartz-phyric. Some clasts are mafic in composition, reflected by pseudomorphs of skeletal olivine phenocrysts that contain chromite. Granite and minor hematite clasts are also evident. Most clasts are well rounded, although some are angular. It is typically clast-supported, but locally matrix-supported. The matrix is a sandstone composed of polymictic volcanic fragments with lesser feldspar, chromite and rare quartz and pseudomorphs of olivine. The chlorite- or hematite-altered matrix has a patchy green or purple colour respectively (McPhie et al. , 2016).
• VBx - polymictic volcaniclastic breccia, which is a hematite-rich breccia composed of porphyritic felsic volcanic clasts (Ehrig et al., 2012), although Ehrig and Clark (2020), Ehrig et al. (2022) and Corriveau et al. (2022) state that it contains both felsic and mafic-to ultramafic clasts from the Gawler Range Volcanics, all set in a 'sea of hematite' matrix.
The first three of these facies, namely KASH, KHEMQ and VASH, are interpreted to have been deposited in a predominantly low-energy, below wave base, subaqueous environment (McPhie et al., 2011), whereas KFMU was laid down during a higher energy depositional event. All of the 'bedded clastic facies' have textures that are indicative of local soft sediment slumping prior to final lithification. Zircon grains from the 'bedded clastic facies' have age populations around 1600 Ma (McPhie et al., 2016). It is considered likely (McPhie et al., 2016) that all of these facies were deposited in a fault bounded sedimentary basin that was active when the breccia complex formed and the hydrothermal system was active.
A further clastic facies has been encountered, but as of 2017, had only been intersected in two drill holes. It is an unaltered, well-sorted, quartz-rich sandstone with faulted contacts and contains zircon grains dated at ~1590, ~1740, ~1900 and ~2500 Ma. This zircon age spectrum is identical to that of the Pandurra Formation, implying that the breccia complex was reactivated, or deformed, after 1424 Ma, the diagenetic age of the Pandurra Formation (Ehrig et al., 2017). However, it seems likely that sections of the Pandurra Formation may be as old as 1575 Ma. This intersection is also predominantly brecciated, with a hematitic sand matrix as described below, but probably post-dates the main Olympic Dam Breccia Complex event.
Alkaline ultramafic to mafic lavas, dykes and possible sills comprise ~30 vol.% of the Olympic Dam Breccia Complex, including those interpreted as the protoliths of heavily hematite altered rocks (Ehrig, 2022, from unpublished mass balance calculations). These include:
Ultramafic volcanic units, occurring as remnants of what are now intensely altered chromite-bearing, olivine-phyric picrite. They are found immediately below the unconformity with the Neoproterozoic cover sequence in the southeastern quadrant of the deposit, adjacent to the 'bedded clastic facies' occurrences. These remnants vary from 15 to 45 m in thickness in eight drill holes, and may represent either a single, or multiple units. The picrite is juxtaposed with various hematite-rich breccias, separated by intensely altered and sheared contacts. Whilst it is strongly carbonate-hematite altered, it has a well-preserved porphyritic texture comprising evenly distributed, skeletal, ~1 mm diameter, relict olivine phenocrysts, and up to ~1 cm wide carbonate-hematite filled amygdales. Carbonate-hematite veins that are 1 to 5 mm in width are also present. Additional diagnostic properties of picrites include elevated concentrations of >0.1% Cr, 100 to 200 ppm V and 300 to 500 ppm Ni. On the basis of its location and texture, this picrite is interpreted to have been a lava (Ehrig et al., 2012).
Pre- to Syn-brecciation Dykes. A suite of mafic and felsic igneous intrusions, mainly dykes, are interpreted to have been emplaced just prior to or during brecciation and mineralisation. The mafic dykes are generally steeply dipping, intruding the Roxby Downs Granite, 'bedded clastic facies', and Olympic Dam Breccia Complex breccias. Some are mineralised, and are both syn-breccia and syn-mineralisation. Multiple generations of dykes commonly intrude along the same structures. Four types are distinguished, based on composition and alteration (after Ehrig et al., 2012), namely:
• Aphanitic, sericite-hematite-altered, probably mafic to ultramafic, dykes, which have been altered to microcrystalline sericite and chlorite and commonly hematite. These dykes, which are best exposed in the deeper parts of the northwestern ore zone, vary from <0.5 up to >40 m in thickness and have inferred strike lengths of as much as 1 km. The contacts with granite-rich breccias are sharp at depth and become more gradational with decreasing depth. They contain micron sized sericite and crystals of zircon, rutile, REE-bearing minerals xenotime, bastnäsite and florencite, and have a characteristic 'waxy' sericite feel/texture. As such, they are anomalous in Ti (>0.3 wt.%), Mg (13 wt.%), Zr (>400 ppm), Al (>9 wt.%) as well as containing other HFSE (e.g., Hf, Th, Ta, Nb), U and K (Ehrig et al, 2022). Intense hematite alteration is common, in which case they are mapped as HMBx. High grade sulphide concentrations are often associated with the margins of, or within these dykes (see Fig. 2 and cross section to the NW of the Masher's Fault on Fig. 3). The chemical composition is interpreted to suggest a mafic to ultramafic protolith, possibly lamprophyre (Ehrig, 2022). They appear to be closely associated with the introduction of mineralisation.
• Sericite-altered, olivine-phyric mafic dykes, which although generally strongly altered, retain a relict porphyritic texture as pseudomorphs after olivine phenocrysts in a very fine groundmass. Olivine appears to have been the only phenocryst in the protolith, and its strong abundance suggests these dykes may have been ultramafic in composition. They are a few to a few tens of centimetres thick and have sharp contacts with the granite and more irregular, lobate or brecciated contacts with the hematite-rich breccia. The contacts are marked by ~3 mm wide glassy zones within the dyke, and ~5 mm wide oxidised rims in the granite.
• Sericite ±chlorite ±hematite-altered, plagioclase-pyroxene-phyric basaltic dykes, which range from relatively fresh to strongly chlorite ±sericite ±hematite altered. Phenocrysts are generally 1 to 2 mm across and difficult to differentiate from the groundmass when strongly altered. These dykes are generally steeply dipping, and at 1 to 5 m thick are relatively narrow, although locally widening to tens of metres. They may occur as cross-cutting, green chlorite-rich dykes that also contain disseminated pyrite and chalcopyrite, and veins of chalcopyrite, pyrite with fluorite and barite. They have a similar composition and texture to the Gairdner Dykes.
• One intensely altered, quartz-phyric felsic dyke has been intersected at a depth of ~1900 to 2300 m in the deepest vertical drill hole (RD2773) in the southeastern part of the Olympic Dam Breccia Complex. It is composed of melt inclusion-bearing quartz phenocrysts in a groundmass of quartz and sericite with minor zircon and apatite, interpreted to imply an original rhyolitic composition. It intrudes weakly brecciated, chlorite-altered Roxby Downs Granite, has brecciated margins and is steeply dipping (Ehrig et al., 2012). Preliminary laser ablation inductively coupled plasma mass spectrometry (LAICP-MS) zircon dating returned an age of ~1590 Ma. On the basis of the intensity of alteration and the contact relationship with the Roxby Downs Granite, it is interpreted to probably be slightly older than the granite (Ehrig et al., 2017).
Johnson and McCulloch, (1995) estimated the ultramafic to mafic rocks accounted for >13 vol.% (~30 vol.%; Ehrig, 2022) of the breccia complex, but contained ~50% of the copper in that complex. Huang (2016) demonstrated that these ultramafic to mafic rocks contain extreme concentrations of some components (e.g. up to 26 wt.% CO2, 50 wt.% of Fe2O3 and 6000 ppm Cr) and 'extraordinary' near linear positive correlations between Cr and high field strength elements such as Ti, Nb and Zr, which is taken to indicate significant whole-rock mass and/or volume loss due to hydrothermal alteration and hence a close association with mineralisation. See the Mineralisation section below for more detail on the role of these mafic to ultramafic dykes.
ODBC - Olympic Dam Breccia Complex
The ~50 km2 and 500 to >1000 m thick Olympic Dam Breccia Complex was principally developed within the Roxby Downs Granite, and to a lesser extent within the Gawler Range Volcanics and bedded clastic facies, with contributions from hydrothermal processes. The degree of alteration and intensity of brecciation increases from the edges of the complex toward the centre of the deposit, with the breccia composition and textural characteristics being gradational. As such, most rocks within the Olympic Dam Breccia Complex form a continuum, ranging from weakly sericite-altered Roxby Downs Granite, to sericite-altered granite-rich breccias, through to hematite-dominant breccias in the deposit centre. The subdivision of the breccias of the complex, based on primary rocks is complicated by the intense hydrothermal alteration, although on a deposit scale, compositional changes across the breccia continuum are systematic and symmetric. The breccias are classified according to the total concentration of iron oxide minerals with clast composition qualifiers. Breccias range from <5 to >90% iron oxides (Ehrig, McPhie and Kamenetsky, 2012) with corresponding whole-rock iron concentrations of up to ~68%.
The texture, contact relationships, distribution and non-stratified nature of the breccias of the complex are interpreted to be consistent with subsurface fragmentation by a combination of tectonic and hydrothermal processes (Oreskes and Einaudi, 1990; Reeve et al., 1990). Further, angular or ragged, amoeboid, and digitate clast shapes suggest both brittle failure and dissolution of preexisting wall rock were important in the generation of these breccias (McPhie et al., 2011).
Ehrig and Clark (2020) describe the evolution of the breccia as commencing on the periphery of the complex as granite with fractures paralleling the NE-SW and NNW-SSE fault pattern (see Fig. 1 above and Structure section below) on a metre to tens of cm spacing, forming fracture bounded rhomboidal blocks of granite. Stepping in towards the deposit, the granite becomes more fragmented with closer spaced fracturing. These fractures are the focus of hematite-sericite alteration, replacing the margins of the rhomboidal granitic blocks and rounding their angular corners at fracture intersections. As this process progresses, the granite blocks become more intensely fractured, and are reduced in size by hematite-sericite alteration replacing the granitic blocks/clasts inwards towards their core. This produces a replacive breccia with sub-angular clasts in a hematite-sericite matrix. As the brecciation and alteration progresses, and the amount of matrix increases, clasts from higher in the sequence, such as the Gawler Range Volcanics and 'bedded clastic facies' are found in the breccia, suggesting the highly corrosive fluorine-rich hydrothermal fluids have dissolved and reduced the volume within the breccia complex, allowing 'solution-collapse' and formation of heterolithic breccia. Towards the centre of the deposit, higher grade Cu mineralisation and fluorine content prevail, where the hydrothermal processes have dominated the initial physical fault controlled brecciation, the hematised granite clasts and remnant quartz grains are sub-rounded to rounded, with the latter being cloudy, while many of the clasts are composed of other hematised lithologies and multiple generations of breccia and hematite.
The principal breccias developed within the Roxby Downs Granite have been divided on the basis of Fe content by Ehrig (2019, 2021, 2022), from the periphery (and at depth) to the upper core of the complex, into:
i). GRN - altered, weakly brecciated granite, to granite-rich breccia, which is biotite-free, and contains <5 wt.% Fe;
ii). GRBx - granite, to hematite-rich breccia, with 5 to 25 wt.% Fe;
iii). HMBx - hematite-rich breccia, with >25 wt.% Fe;
iv). HEMQ - hematite-quartz-barite breccia, with >60 wt.% Fe;
The geological description of the transition these divisions represent was best described by Reeve et al. (1990), a summary of which is as follows:
Brecciated Granite to Granite-Rich Breccias, which span a wide range of textures that reflect variable intensities and styles of brecciation and fragment transport, ranging from sparsely fractured or veined granite on the margins of the complex, to coarse clast supported breccia with rotation of blocks/clasts inward. Crackle and jig-saw textures are commonly preserved in matrix poor granite breccias, particularly in less altered zones. These textures become progressively less recognisable as the proportion of matrix increases. Apart from superimposed alteration, the clast lithology is indistinguishable from Roxby Downs Granite external to the complex. This transition is interpreted to comprise a replacive breccia, where the fracture controlled veining/alteration expands, consuming the granite wall-rocks which remain as progressively smaller preserved kernels, and when isolated in the alteration matrix may rotate.
Some of the granite-rich breccias are composed of isolated large clasts of granite that are up to tens of cm across, supported by a finer grained, variably altered, granite-derived matrix. In these breccias, the matrix may show some sorting, with locally developed discontinuous and diffuse layering, and some local, irregular, matrix-rich micro-breccia patches resembling arkoses or diamictite-like fabrics. The bulk of the clasts and matrix fragments are angular to subangular, and subequant to elongate in shape, with only rare instances of substantial rounding in either. However, where there is an intense sericitisation or silicification alteration overprint, clast boundaries are generally highly irregular or diffuse.
Breccias with granite fragments within a hematite rich matrix are part of the continuum to hematite-rich breccias and locally contain abundant sulphides. These breccias range from matrix poor granite breccias containing hematite rich crackle veins, through more matrix rich transitional varieties, to breccias dominated by a hematite-quartz ±sericite/muscovite matrix containing minor barite and fluorite. Some contain cataclastic fabrics, whilst others have unusual fabrics suggestive of both ablation and attrition.
Hematite-Rich Breccias are extensively developed, and the most important host to mineralisation. These have been subdivided into three broad, transitional breccia types, as described individually below. However, all three have common textural features, as follows:
i). fabrics range from matrix to clast rich, although the former are generally more abundant;
ii). the clast size is very variable, and although isolated blocks up to metres, or rarely tens of metres in diameter occur, most are generally <20 cm;
iii). although local zones of limited sorting have been observed, most breccias are poorly sorted;
iv). clasts may be locally predominantly irregularly shaped, but are usually angular and approximately equi-dimensional;
v). substantial rounding of clasts is rare, even where the presence of heterolithic breccias suggest remobilisation and mixing;
vi). repeated brecciation is indicated in many of the breccia clasts and bodies.
The spectrum of hematite-rich breccias includes:
• Heterolithic Hematitic Breccia - a broad grouping that includes the greatest proportion of hematite-rich breccias at Olympic Dam, and hosts the bulk of the Mineral Resource. It is predominantly composed of a range of hematite clasts, but may also contain a significant proportion of altered granite fragments, with the amount of each clast type being highly variable. Those clasts that are mostly hematite-rich, range from fine-grained and dark red-brown, to fine- or medium-grained crystalline black varieties. Less commonly, these clasts include hematite that has a coarse feathery-texture and is crystalline, brick-red and black powdery, or dense steely grey. The strongly heterolithic nature of these breccias suggest they have been subjected to a degree of mixing, and possibly significant transport. Never-the-less, these heterolithic hematitic breccias may contain poorly defined 'pockets' that are metres to tens of metres across where similar clast types dominate, or in which the granite clasts have the same composition and alteration type. Granite clasts of conspicuously different alteration style are rarely found in very close proximity.
Other clast lithologies, though minor, contribute to the heterolithic nature of these breccias, and include highly altered, fine grained, mafic and felsic igneous intrusive material, finely laminated sedimentary rocks that are predominantly composed of hematite and quartz, and massive to diffusely layered medium to coarse grained arkose-like rocks containing altered granite-derived feldspar and quartz fragments. Some of these breccias also contain minor clasts of massive chalcopyrite, barite, fluorite or siderite. Localised breccia zones near the northeastern margin of the deposit include a major proportion of siderite clasts.
The matrix of the heterolithic hematitic breccias is normally dark red-brown to black, fine- to medium-grained hematite, with a variable amount of quartz and other material largely derived from granite. Commonly there is an irregular 'streaky' mixture of dark red-brown and black hematite that may define a crude layering. Some of these layers may also have undergone limited sorting, particularly among the non-hematite grains. Copper sulphides, pyrite, fluorite and barite may also be disseminated in the matrix.
• Hematite Breccia, in which both the clasts and matrix are largely composed of hematite. Although this breccia type is the least abundant of the 'hematite-rich breccias', it may be the most strongly mineralised, preferentially containing the highest grade ore, particularly in the northwestern parts of the deposit. However, where developed well below the bornite-chalcopyrite interface, lower in the mineralised system, it commonly contains abundant pyrite and only minor copper sulphides.
Hematite breccias display a wide variation in colour, texture, and composition of the minor non-hematite component. The breccia is generally dark grey to black, reflecting the predominance of hematite grains with well developed crystal forms. They are composed of fine- to medium-grained, obvious crystalline hematite with interlocking mosaic, tabular or bladed textures, and have a distinctly contrasting appearance to the other two hematite-rich breccia types in which pulverulent, earthy or dusty red-brown to purplish hematite is more common. These black to grey hematite breccias range from dense and hard, to very porous or vuggy types, with extremely porous varieties containing up to ~30% voids. Some hematite breccias contain abundant small vugs which appear to be the result of leaching of sulphides. Some are only weakly brecciated, or appear to have been brecciated in situ, although in some cases, there is only very subtle textural differences between the clasts and matrix and they are difficult to discern.
Non-hematite components are variable, and may include quartz, chlorite, fluorite, barite, altered granite clasts and granite-derived mineral fragments, siderite, minor relict magnetite and sulphides ±pitchblende in mineralised areas. Reeve et al. (1990) note that minor amounts of hematite breccia may contain distinctive components and/or exhibit unusual textures, including hematite-rich or hematite-sulphide pisolites, strongly laminated hematite rich rock fragments, wispy discontinuous layering, crustiform hematite, and coarse feathery-textured hematite. These may occur as individual isolated clasts within hematite breccias, or as small 'pockets' of particular clast types.
• HEMQ - Hematite-Quartz Breccia is the most intensely altered rock type within the Olympic Dam Breccia Complex. It is composed of clasts of hematite, quartz and hematite-quartz set within a matrix that is predominantly hematite with grains of quartz. It is texturally destructive and resulted in Al depletion corresponding to the removal of all aluminosilicate minerals. It is largely restricted to the shallow central core of the breccia complex, although minor amounts of similar breccias are found elsewhere within the deposit. This breccia, which is predominantly in the hanging wall of the ore, is characterised by abundant quartz fragments, particularly in the matrix, where they may constitute as much as 40% of the rock. Reeve et al. (1990) note that the crystal morphology and characteristic undulose extinction of this quartz indicates it was derived from the local granitic lithologies, although fragments of vein quartz and/or a range of other strongly siliceous or silicified rocks are locally abundant. However, Ehrig et al. (2021) suggest the bulk of the HEMQ is after lithologies of the Bedded Clastic Facies.
The HEMQ typically contains ≥110 ppm As, <2.5 wt.% Al and < 0.35 wt.% Cu (Ehrig and Clark, 2020).
The Hematite-quartz breccia is also characterised by dominant fine grained dusty red-brown to dark purplish hematite in both clasts and matrix. Steel-grey to black hematite clasts are also present, although much less abundant and generally smaller than the coexisting red-brown or purplish types. It is also noted for the abundance of barite, generally 2 to 5%, occurring as disseminations, veins and vein fragments, and for the scarcity of fluorite compared to other hematite-rich breccias.
Post-brecciation Rock Units
KGRN - Pandurra Formation, which regionally which was deposited in the ∼42 500 km2, ~420 x 170 km Cariewerloo red bed basin that unconformably overlies the Gawler Range Volcanics, as described above in the Regional Setting section and the Gawler Craton and Olympic IOCG Province record. However, as described above, two drill holes (as of 2022), have each intersected ~80 m an unaltered, well-sorted quartz-rich sandstone with faulted contacts which contains a spectrum of detrital zircons identical to those of the Pandurra Formation. It has therefore been interpreted to represent a slab of Pandurra Formation overlying the 'bedded clastic facies' which has been structurally interleaved with the latter and down-faulted into the Olympic Dam Breccia Complex (Ehrig et al., 2017). This sandstone is almost entirely brecciated, with only the uppermost 2.3 m and another lower ~2 m section that appear to be un-brecciated, although both could conceivably be large clasts. The breccia clasts vary from <1 to tens of cm across and range from angular jigsaw fit to separated sub-rounded blocks. They are composed of well sorted, quartz-rich, massive to diffusely stratified sandstone, with some being cross-bedded. The sandstone of the clasts is composed of sub-rounded quartz and mm-sized muscovite grains, with tourmaline and fine-grained red hematitic fragments. The quartz grains have undulose extinction and abundant fluid inclusions, whilst some are poly-grain aggregates with annealed, triple-junction boundaries. The breccia clasts are set in a poorly sorted hematitic matrix that is markedly different in character to the clast lithology, but comparable to the hematite breccias. This brecciated sandstone has a sharp upper and gradational lower contact with polymictic hematitic breccia of the Olympic Dam Breccia Complex. No other drill holes on the same section intersect this unit, with the nearest passing 50 and 250 m to the SW and NE respectively (McPhie et al., 2016).
Gairdner Dykes, which have been dated at between 827 ±6 and 824 ±4 Ma, and are weakly altered, varying from coarse, equigranular dolerite to finely porphyritic basalt. They are composed of equigranular pyroxene and plagioclase crystals that are intergrown in the dolerite, or occur as phenocrysts in the porphyritic basalt. Magnetite is responsible for their characteristic magnetic susceptibility of ~>100 x 10-3 SI units. Multiple generations of dykes are evident in some cases, with early dolerite being intruded by later porphyritic basalt. Contacts between these dykes and the Roxby Downs Granite or Olympic Dam Breccia Complex are sharp and marked by perlite or palagonite-altered glassy margins typically a few millimetres thick. In some instances, the dolerite may be weakly chlorite altered with incipient hematite alteration at shallower levels, whilst narrow carbonate veins are common. Although weakly altered, this dolerite is not mineralised. It is characterised by Cr, V, Ni and Ti contents of 50 to 100 ppm, 300 ppm, 50 to 100 ppm and 1.5 to 2 wt.%, respectively.
Neoproterozoic to Cambrian sedimentary rocks, that form the cover sequence that comprises ~350 m of flat-lying, shallow marine shale, quartzite, and dolostone, unconformably overlying the sub-horizontal eroded upper surface above the Roxby Downs Granite, Olympic Dam Breccia Complex and Mesoproterozoic sedimentary sequences.
STRUCTURE
A complex array of brittle faults are seen within and surrounding the deposit. There are interpreted to include pre-, syn- and post-mineralisation structures (Clark, Passmore and Poznik, 2017). Ehrig et al. (2017), however, note that annealing by intense alteration overprinting of pre- and syn-mineralisation structures hampers the easy recognition of these faults. Never-the-less, the same authors suggest the NW strike of ore zones in the north-western part of the deposit, sharp geochemical fronts and evidence of multiple brittle deformation events within the breccias are strong indicators of pre- and syn-mineralisation structures. Post-mineralisation faults are evident in the central section of the deposit where they disrupted primary mineralisation trends. In addition to numerous local-scale structures, five main fault sets are recognised in and around the deposit, all of which are steeply dipping. However, rotation related to late stage faulting may have changed the orientation of earlier structures. It should also be noted that some of the earlier trends are not obvious on Fig. 1 as they are not major structures. Ehrig et al. (2012) described each of the fault sets as follows:
• North-south faults, that are generally discontinuous and offset by NW, NE and WNW sets, although there is some suggestion of late reactivation. The longest mapped faults of this set extends for over 1 km. Enrichment of hematite and copper is associated with this trend, whilst 'bedded clastic facies' blocks and pre- to syn-mineralisation dykes are located at the intersection with WNW faults.
• NW-SE to NNW-SSE faults reflect a trend that is regionally continuous (e.g., the Elizabeth Creek fault zone of Hayward and Skirrow, 2010 which is ~40 km to the SW). These faults are offset by NE structures and by Masher's Fault. Within the deposit, these structures are associated with hematite alteration, copper mineralisation, pre- to syn-mineralisation mafic dykes and late post-mineralisation dolerite intrusions. There is some evidence of late reactivation.
• WNW-ESE to east-west faults, that are regionally discontinuous and appear to splay from NW faults in the vicinity of NE structures, although they may also be slightly earlier than the NW faults. Hematite alteration, copper, and locally molybdenum mineralisation are associated with these structures where they occur in the immediate hanging wall of NE faults. There is evidence of minor reactivation, which is intensified closer to Masher’s fault with sinistral offsets. In the deposit area, this trend is represented by the Woodall Fault and Woodall Fault Junior, ~400 m to its north, which both dip steeply to the NNE. These faults together, have been estimated to represent a 2.5 to 3 km of north-side down normal offset. They have been responsible for the relative uplift of the Roxby Downs Granite and downthrow and tilting to near vertical of the 'bedded clastic facies', and probably persisted until after the deposition of the Pandurra Formation on its northern side. This displacement brings the unaltered Roxby Downs Granite in much closer proximity to the centre of the deposit than elsewhere. This downthrow has also been responsible for the deepest occurrence of mineralisation, with drill holes terminating in grades of >2% Cu and 1.5 g/t Au at depths of >1750 m depth (Ehrig and Clark, 2020). Abundant 1 to 5 mm thick quartz-tourmaline, quartz-carbonate and quartz dominant veins, which are rare elsewhere within the deposit, do occur between the Woodall and Woodall Jr faults. In addition, both ~1590 Ma mafic to ultramafic and ~825 Ma Gairdner dykes intrude along both of these faults suggesting long lived activity from at least 1590 to 1424 Ma. Ehrig (2022) queries whether the Woodall and Woodall Junior faults are an annealed 'mega-shear' as although displacement is indicated, no well defined fault plane has been intersected in drilling.
• NE-SW to ENE-WSW faults, which are also regionally continuous (e.g., the Todd Dam fault zone; Hayward and Skirrow, 2010) with the most recent displacement being sinistral. They offset NW, WNW and north-south fault sets. Some are markedly vertical and penetrative, and control the emplacement of mafic dykes. Others are now listric, e.g., the Oreskes Fault, which represents an original major domain boundary that was a normal fault during development of the deposit. Where NE-SW and WNW faults intersect, they are associated with both hematite alteration and copper mineralisation. Regionally they are mapped as being reverse structures, although at the deposit scale, there is evidence to suggests normal displacement. Where they are steeply dipping, they have been strongly reactivated in association with the sinistral movement on the major Masher's fault, described below, to which they are parallel to sub-parallel.
The prevailing structural architecture in the southeastern lobe of the deposit is predominantly composed of a series of east- and west-dipping normal faults with a NE-SW orientation. These faults splay from, or displace, the Woodall and Woodall Junior faults with normal offsets of tens to hundreds of metres. Together with the Woodall Junior and Lalor faults to the south and north respectively, these faults fundamentally controlled the initial structural dismemberment of the deposit and incorporation of the allochthonous fault dislocated, ~1.5 x 0.6 x 2 km block of 'bedded clastic facies' into the Olympic Dam Breccia Complex (Fig. 1; Clark and Ehrig, 2019).
• Listric extensional faulting is evident within the deposit area, occurring as moderate to shallow to flat lying normal faults (Fig.s 2 and 3) that mark some lithological boundaries (e.g., the base of the HEMQ breccia on Fig. 3). These structures appear to be mostly post-mineral, with many being truncated by late steep NE-SW and north-south structures, although others (Fig. 2) appear to be the down-dip sections of some of the NE-SW faults that are steeper at surface. However, some of these listric faults appear to parallel shallow dipping breccia zones and high grade mineralised ore shoots, and may represent reactivated earlier extensional structures.
• Masher's fault, the local mine name for the regional Jubilee fault zone (Hayward and Skirrow, 2010) which is a major zone of crustal weakness. Regionally it has an ENE trend, and sinistral displacement, but in the deposit area parallels the NE-SW trending structures and is a 150 m wide zone of faulting. During the history of the Olympic Dam deposit it has been reactivated on several occasions. Typically, whenever there has been a major deformation event within the Gawler Craton, hydrothermal modification and structural dislocation is recorded in the deposit, centred on the Masher's Fault zone, and from there, distributed through its subsidiary structures. The main body of the deposit is centred on the Masher's Fault intersection with the postulated pre-mineral NNW-SSE (to NW-SE) fault that is inferred to control the main elongated mineralised zone to the NW. It does not appear to have influenced the original development of hematite breccias or copper mineralisation, but does markedly truncate and offset both lithofacies, alteration and mineralisation. In the deposit area, south side down normal displacement has preserved substantial fault blocks of 'bedded clastic facies' in the southeastern lobe of the deposit, juxtaposed against 'deeper' brecciated and mineralised Roxby Downs Granite to the NW of the fault. It was active as late as the Cambrian, displacing the Early Cambian Andamooka Limestone in the overlying cover sequence and is reflected by a ~100 km long regional aeromagnetic feature.
OD DEEPS ZONE
The OD Deeps Zone is a steeply dipping, structurally controlled downward extension of the main, 6 x 3 km, grossly tabular and flat lying, Olympic Dam main resource that generally extends no more than 800 m below the unconformity with the cover sequeunce. It lies below the southern margin of that resource envelope, and is located to the SE of the long-lived, major, Masher's Fault (Figs. 1 and 5). The OD Deeps zone is largely fault bounded, particularly to the south by the Woodall Fault complex, which is defined by the Woodall and Woodall Jr. faults (Fig. 4, Section G-H). Ehrig et al. (2024) infer that at least in the west, it is also bounded to the north by structures such as the Lalor Fault (Fig. 4, section G-H). Mineralisation extends for ~2.5 km along strike and over a vertical interval in excess of 1 km to a depth of >2 km, and thickness of ~150 to 300 m. It appears to taper to the east, both down dip and along strike, and is open down dip (Fig. 5). To December 2023, the zone had been tested by 62 km if drilling, mostly wedged drill hole spreads from surface. Drill spacing ranged from 160 to 320 m, with 28 intersections demonstrating continuity of >1% Cu mineralisation. The mineralisation intersected is similar to that of the main Olympic Dam deposit, the base of which is generally shallower than 1 km in depth. However, in contrast, the copper sulphide mineralogy is dominated by chalcopyrite, although some areas of bornite are encountered, mainly towards the upper overlap with the main resource. Intersections to the west include the oblique, partly down-plunge drill hole RU50-12091 that cut 580 m @ 2.2% Cu, 473 ppm U3O8, 0.93 g/t Au, 4.87 g/t Ag, including 126 m @ 4.41% Cu, 945 ppm U3O8, 0.99 g/t Au, 7.13 g/t Ag (Fig. 5, Section J-K). Other holes cutting across the ore zone on the same section, include intervals of >100 m of >2% and/or 1 to 2% Cu, e.g., RD4552AW2 with 390 m @ 1.38% Cu, 396 ppm U3O8, 0.65 g/t Au, 2.43 g/t Ag, including 52 m @ 1.72% Cu, 483 ppm U3O8, 0.63 g/t Au, 1.33 g/t Ag.
On the eastern extremity of the zone (Fig. 5, Section L-M), intersections include drill hole RD4551W1 with 172 m @ 1.52% Cu, 348 ppm U3O8, 0.65 g/t Au, 7.91 g/t Ag, including 50 m @ 2.03% Cu, 355 ppm U3O8, 0.83 g/t Au, 9.11 g/t Ag.
Fig. 4 (section G-H) is an idealised cross section through the OD Deeps zone from 1 to 2 km depths, whilst the smaller scale Section E-F in Fig. 4 overlaps the transition from the main deposit, to the top of the OD Deeps mineralisation. Fig. 5 illustrates the relationship between the main resource model and the drill intersections of the OD Deeps zone not yet included within that model.
Over much of the Olympic Dam deposit, there is a broad, diffuse transition from the intensely hematite altered core of the mineralised system, outward and downward through weakly altered (<5 wt.% Fe) to unaltered Roxby Downs Granite (Fig. 1). In contrast, on the southern margin the OD Deeps zone, this transition is telescoped to a few hundred metres across the composite Woodall Fault complex. Ehrig (2024) suggests this is a reflection of post-mineral faulting.
OD Deeps mineralisation is hosted by both strongly developed hematite altered breccias where the protolith is completely obliterated, encroaching upon a host that includes both granite and rotated, down-faulted blocks of less altered volcanics and Bedded Clastic Facies. Whilst as detailed below, the mineralising system at Olympic Dam was altering and mineralising the Roxby Downs Granite at depth prior to the Bedded Clastic Facies, it would seem that the same alteration and mineralisation progressed upward into those facies contemporaneously with their rotation and down-faulting into an extensional graben. However, it is also evident that the down-faulting continued or was reactivated well after the initial mineralising system was dormant.
This OD Deeps summary is drawn from a presentation to the AusIMM by K. Ehrig in February, 2024 and the BHP Operational review for the half year ended 31 December 2023.
Click here for cross section location map
ALTERATION
Skirrow (2022) notes that regionally, Na-Ca-Fe metasomatism is represented in the Olympic IOCG Province by assemblages of hydrothermal albite-calcsilicate comprising actinolite and/or diopside ±magnetite, commonly accompanied by minor titanite and scapolite. This alteration typically forms kilometre-scale regional alteration zones in both the Mount Woods and Moonta-Wallaroo districts, well to the north and south of Olympic Dam respectively. However, sodic alteration is much less evident in the Olympic Dam district where rarely observed hydrothermal albite is suspected to represent relicts of sodic metasomatism surviving after widespread K feldspar overprinting (Bastrakov et al., 2007). This Fe2+-K metasomatism is represented by magnetite-K feldspar alteration. Relative timing relationships indicate that regionally, albite-actinolite ±magnetite alteration is overprinted by a biotite-magnetite assemblage, which, in turn, is replaced and cut by the hematite-sericite-chlorite-carbonate alteration typical of Olympic Dam (Skirrow, 2022).
The principal hydrothermal alteration minerals at Olympic Dam include hematite, magnetite, muscovite (1M and 2M1 types) and quartz, with lesser chlorite (chamosite/chlinoclore), siderite, fluorite, barite, paragonite, albite and K feldspar (Oreskes and Einaudi, 1990; Reeve et al., 1990; Ehrig, McPhie and Karnenetsky, 2012; Mauger et al., 2016; Kontonikas-Charos et al., 2017; Ehrig et al., 2017).
Iron oxide alteration represents: i). a progressive spatial increase in Fe from edge and depth to centre and top; and ii). a temporal shift from the more reduced Fe2+ → the more oxidised Fe3+, represented by magnetite + apatite + chlorite → hematite + sericite. Another zonation from edge to upper-core is represented by siderite → fluorite → barite.
There is a complex distribution of alteration minerals throughout the hydrothermal system, although deposit-scale alteration assemblage trends are evident. Beginning from the periphery, alteration is first seen in the Roxby Downs Granite, distal to the Olympic Dam Breccia Complex. The outer margin of the breccia complex is marked by the boundary between the external 'unaltered' and the weakly altered Roxby Downs Granite. This boundary has been defined to be at the 'biotite-out' contour, internal to which no magmatic biotite remains. However, this alteration change, which is 1 to 5 km from the deposit centre, is transitional, with external incipient sericite/muscovite alteration of plagioclase, while igneous biotite is progressively converted to chlorite, until no biotite remains at the 'biotite-out' contour.
Alteration then progressively increases in intensity inward within the Olympic Dam Breccia Complex, where iron metasomatism is much higher and hydrothermal K feldspar is replaced by muscovite.
Three major deposit-wide zoned alteration assemblages and one minor style are recognised (Reeve et al., 1990; Ehrig, McPhie and Kamenetsky, 2012; Ehrig et al., 2017; Ehrig and Clark, 2019; Corriveau et al., 2022), namely:
i). Paragenetically early, relatively reduced, Fe oxide alteration, characterised by high temperature (~800 to 400°C) magnetite-fluorapatite-ankerite-calcite/siderite-chlorite-quartz, which occurs at depth and outward in the deposit on the periphery of the mineralised areas with ~5 wt.% Fe. This early alteration, referred to as the 'footwall distal alteration' assemblage, involved the development of a high temperature, iron-rich, Ca-Fe alteration (i.e., an early IOA style mineralisation) that evolved to high ƒCO2 conditions, low calcium contents and crystallisation of siderite and chlorite. This zone has an elevated magnetic susceptibility compared to the rest of the deposit, reflecting the relict magnetite being progressively overprinted by siderite/ankerite and later by hematite inwards and upwards into the mineralised system. It also corresponds to the chalcopyrite-pyrite mineralised zone which typically has <1% Cu, as described below in the Mineralisation section. This transition is reflected by chalcopyrite-pyrite mineralisation cutting early high temperature Ca-Fe magnetite-apatite alteration. This is subsequently brecciated and infilled by calcite, while being cut by chlorite-sericite-chalcopyrite and pyrite, as preserved in the deeper, peripheral part of the Olympic Dam Breccia Complex. The siderite and then hematite (after magnetite) formed as the hydrothermal system evolved with a chalcopyrite-pyrite mineral assemblage overprinting the earlier high temperature Ca-Fe facies. Hyper-saturation of CO2 led to an influx of siderite-stable alteration, accompanied by hematite alteration of earlier high temperature Ca-Fe facies and deeper calcite alteration. Siderite also occurs as hydrothermal infill and cement within syn-mineralisation fault zones.
Drilling on the eastern margin of the complex, external to the mineral resource, to a depth of ~2330 m, encountered this assemblage with minor disseminated chalcopyrite, sericite, chlorite, rare earth element-fluorcarbonates, monazite, uraninite, thorite, galena, sphalerite, anhydrite, schorl, rutile and pyrrhotite, cut by abundant multiphase veinlets and calcite ±fluorite ±barite veins (e.g., Apukhtina et al., 2017). Ehrig et al. (2017; 2010) show siderite occurring as a well defined zone at depth and as a 'patchy' halo surrounding the main deposit at shallower levels, overlapping and passing inward into the zone of greater fluorite concentration. The siderite also closely corresponds to the distribution of K feldspar, although it is uncertain from the literature how much of the latter is an alteration assemblage, as distinct from original magmatic feldspar in the Roxby Downs Granite that has not been altered to chlorite and/or muscovite. The siderite and K feldspar appear to underlie/flank the high grade Cu and U3O8, but overlap a substantial amount of the lower grade underlying and outer peripheries of that mineralisation.
ii). The Fe2+ stable early siderite/ankerite and magnetite-bearing assemblage is replaced by a low temperature, more oxidised Fe3+ K-Fe and Ca-Fe facies, characterised by the assemblage of hematite-muscovite-quartz-fluorite. This alteration assemblage is referred to as the 'ore zone alteration'. The transition is typically sharp and associated with a marked increase in sulphide content, including bornite-chalcocite mineralisation. This assemblage progresses from the poorly brecciated, weakly hematite altered Roxby Downs Granite overlapping the siderite/magnetite zone along the margins of the Olympic Dam Breccia Complex, through increasing muscovite-hematite, into intense hematite-muscovite, to dominantly hematite alteration. This hematite-rich assemblage is the principal host to the economic sulphide mineralisation, with the Fe2+/Fe3+ redox boundary broadly coinciding with a sulphide mineral zoning transition from chalcopyrite to bornite-chalcocite assemblages. The latter transition varies from being 'knife-sharp' to tens of metres wide. This boundary also corresponds to an increase in fluorite which accompanies most of the bornite-chalcocite mineralisation. The latter constitutes the bulk of the high grade ore which also straddles the transition outward into the chalcopyrite zone (Ehrig and Clark, 2020).
An inner marginal zone of muscovite, illite ±hematite ±fluorite alteration is enriched in gold and lead, which is interpreted to represent later, post-mineralisation remobilisation, spatially controlled by second- and third-order structures in favourable alteration-protolith sites (Corriveau et al., 2022).
iii). An end member assemblage of hematite-quartz-barite, with no sulphides is formed by the complete conversion of muscovite to hematite. This includes the replacement of all aluminosilicates (including chlorite and muscovite) in the upper core of the deposit, and in fault limited smaller zones along the north-west arm of the deposit. This is an end-member alteration type where the original protolith texture has beeen almost totally obliterated, and virtually completely replaced by hematite. This stage includes Ba-Si-Fe and Si-Fe facies, both of which are deposited at low temperature, and is referred to as ‘hanging wall-type alteration'. This assemblage reflects another redox boundary between S1- sulphide bearing assemblages to SO42- sulphate stability. Hematite and quartz occur as clasts within a matrix of hematite and quartz grains, with minor fragments of vein quartz and/or a range of other high siliceous or silicified rocks being abundant locally (Reeve et al., 1990). Barite occurs as disseminations, vein fragments and abundant narrow, <1 mm barite veins with barium averaging ~2 to 5 wt.% (Reeve et al., 1990). Sulphide mineralisation, which is rarely >0.05 wt.%, is confined to minor late veins and joints. This alteration assemblage has margins that are commonly silicified and barite veined, and may contain gold-only mineralisation (Ehrig, McPhie and Kamenetsky, 2012). Variations and ghosts in the texture may reflect different, extremely altered protoliths (e.g., Roxby Downs Granite, felsic and mafic volcanic fragments after Gawler Range Volcanics, and 'bedded clastic facies'; Clarke and Ehrig, 2019). This alteration assemblage is the HEMQ breccia (described above in the Geology section), which is barren of Cu and U, but may contain Au on its outer margins. It is largely confined to the upper core of the deposit, although other more restricted zones are distributed along the dominant NW-SE axis of alteration and mineralisation (Reeve et al., 1990; Ehrig, McPhie and Kamenetsky, 2012). Its lateral and basal margins are typically faulted, and are interpreted to be preserved as down-faulted blocks spatially associated with the bedded clastic facies. The HEMQ is up to 700 m thick, and extends for from 400 to 750 m below the Neoproterozoic unconformity.
Contiguous zones of high-grade mineralisation follow the margins of the HEMQ. Although the geometry of the HEMQ varies significantly along its strike length, it appears to critically control the distribution of much of the mineralisation, which, if present, wraps around the contact from where it is steep on its lateral margins, to flat-lying beneath the base of the main 'bowl-shaped' HEMQ mass (Figs. 2 and 3; Clark and Ehrig, 2019). Mineralisation is dominantly chalcocite-bornite proximal to the contact, before chalcopyrite is found more distal and at depth. Minor inflections are observed along the margins of the HEMQ, where strongly localised high-grade concentrations are found in 'antiformal' geometries. However, despite this apparent intimate relationship, it should be noted that not all margins of the HEMQ are mineralised, and that a significant volume of high-grade mineralisation is observed distal to its contact (Ehrig and Clark, 2020).
iv). Localised occurrences of paragonite-illite-quartz ±zunyite ±diaspore ±kaolinite advanced argillic alteration remnants that are partially to wholly replaced by hematite.
Distal to the main alteration/mineralised system of the Olympic Dam Breccia Complex, relatively minor post-magmatic alteration of the Roxby Downs Granite takes the form of an evolution from cryptoperthite* to patch perthite*. Patch perthite was subsequently overprinted by high porosity, near end-member albite and K feldspar, whereas plagioclase is partially replaced by albite-muscovite ±Ba-rich K feldspar, both of which are isochemical processes requiring no addition of K and Na from an external source (Kontonikas-Charos et al., 2017). Inward within this zone, biotite and amphibole are progressively altered to chlorite, until no biotite remains at the margin of the breccia complex, whilst magnetite is converted to hematite. Muscovite alteration of magmatic and hydrothermal feldspars and chlorite persists into the margins of the Olympic Dam Breccia Complex, whilst plagioclase is completely altered to muscovite plus albite, which is, in turn, then altered to muscovite (Ehrig et al., 2017).
*NOTE: Cryptoperthite is a fine-grained, submicroscopic feldspar variety that has the structure of perthite, and consists of an intergrowth/interlamination of potassic and sodic feldspar (albite and K feldspar), detectable only by x-rays or an electron microscope. Patch perthite is an intimate intergrowth of sodic and potassic feldspar resulting from sub-solidus exsolution.
The stages that followed the early Fe2+ phase of the footwall, are composed of the following hydrolytic alteration that overprints and largely obliterates early alkali-calcic and potassic alteration. These processes produce assemblages that include hematite, muscovite, phengite, chlorite, quartz, siderite, fluorite, barite and remnants of kaolinite, pyrophyllite and topaz (Ehrig et al., 2011; 2017, Ciobanu et al., 2019, Verdugo-Ihl et al., 2020; Ehrig and Clark, 2020, Ehrig 2022):
• Hematite alteration - Hematite has a history of multiple pseudomorphic replacement of pre-existing lithologies and minerals, accompanied by corrosion, overgrowth and recrystallisation. It replaces and pseudomorphs magnetite, siderite, chlorite; sometimes sulphides, feldspars, fluorite and barite; and rarely as infill in the breccia matrix. In the more heavily altered intervals it occurs as both oscillatory and 'sector-zoned' hematite, with haloes of overprinting porous hematite with corrosive margins between the two. The oscillatory and zoned hematite may be displaced across fractures that are commonly filled with the porous variety. The porous hematite can contain sub-micron inclusions of U and REE minerals that coarsen to µm-scale inclusions as the porosity increases (Ciobanu et al., 2013). Hematite is also precipitated in vugs. Hematite alteration of magnetite is represented by: Fe3O4 + 2H+ → Fe2O3 + Fe2+ + H2O. This conversion is stimulated by a decrease in temperature in higher, cooler, later parts of the system as it degrades from magnetite-stable conditions at temperatures in the range 450 to 350°C at depth, to hematite stability at <350°C (e.g., Corriveau et al., 2022). However, much of the hematite alteration is the result of dissolution of the other replaced minerals and/or substitution by hematite from a hydrothermal solution.
The two broad, peripheral zones of the Olympic Dam Breccia Complex comprise the outer weakly altered brecciated-granite to granite-breccia (GRN) and inner granite- to hematite-rich breccia (GRBx) with <5 and 5 to 25 wt.% Fe respectively, and hence, relatively minor hematite alteration, as described previously in the Geology section (see Fig. 1). The inner granite-rich breccia, passes inwards into narrower, more discrete zones of hematite-rich breccias, defined by the >25 wt.% Fe contour, where hematite becomes the most abundant component. These hematite-rich breccias are also predominantly derived from Roxby Downs Granite, but reflect a greater intensity of brecciation and a higher proportion of hydrothermal constituents, principally hematite, relative to the granite-rich clast breccias. Hematite-rich breccias are the major host to sulphide mineralisation. Hematite may be red-brown to black. Increased hematite alteration of the Roxby Downs Granite coincides with a marked depletion of free silica, from 30 to 35 wt.% Si (65 to 75% SiO2) in the fresh granite, to <2.5% wt.% Si (<5% wt.% SiO2) in hematite breccia with 60 wt.% Fe (Ehrig et al., 2017). This represents a substantial volume of silica that has been leached from the granite, some of which may have been deposited as late veinlets cutting the HEMQ hematite-quartz breccias and as zones of quartz veining on the margin of the deposit.
• Chlorite alteration - Mg-Fe chlorite has replaced magmatic biotite and amphibole in the Roxby Downs Granite, and in mafic-ultramafic clasts in hematite-breccias, as well as the mafic silicates in the abundant mafic-ultramafic dykes, lavas and sills. The intensity of alteration increases proximal to contacts with mafic and ultramafic dykes and intrusions, but grades into/is replaced by, intense sericite altered breccias distal from the contacts with pre- to syn-brecciation dykes. Chlorite alteration appears to increase with depth in granite-derived breccias, typically pseudomorphing feldspars. Ehrig et al. (2017) show the main, relatively narrow sheet of more intense chlorite immediately overlying the siderite-K feldspar zone and passing up into, and overlapping muscovite. Muscovite alteration typically overprints and replaces chlorite. Alteration of biotite to chlorite by an acidic fluid is represented by the equation:
2K(Mg,Fe)3AlSi3O10(OH)2 + 4H+ → (Mg,Fe)5Al(Si3Al)O10(OH)8 + 3SiO2 + (Mg,Fe)2+ + 2K+, releasing silica and potassium (Ehrig, 2022).
• Sericite/muscovite alteration (which with other white micas are collectively referred to as muscovite) - muscovite typically replaces feldspars and chlorite, and occasionally occurs as breccia matrix infill. It is widespread throughout the Olympic Dam Breccia Complex, occurring as an alteration product of igneous sodic plagioclase (albite and oligoclase), igneous orthoclase, and hydrothermal chlorite, and is, in turn, replaced by hematite, culminating in the hematite-quartz-barite breccia. Incipient muscovite alteration of sodic plagioclase occurs in relatively unaltered Roxby Downs Granite, whilst it completely replaces plagioclase when the accompanying Fe concentration reaches 5 wt.%. Similarly, the orthoclase content steadily decreases as the Fe concentration increases, until it essentially disappears to <1 wt.% when Fe becomes >35 wt.%. Clasts of feldspar-phyric felsic lavas in hematite breccias are variably altered to sericite and hematite. Ehrig et al. (2017) show the zone of strongest muscovite alteration immediately underlying and flanking the intense Fe alteration of the HEMQ Breccia, and directly overlying the chlorite zone. However, moderate muscovite alteration continues downward and outward, overlapping both chlorite and the siderite-K feldspar zones. Alteration of alkali feldspar to muscovite by an acid fluid is represented by the equation:
3KAlSi3O8 + 2H+ → KAl2(AlSi3O10)(OH)2 + 6SiO2 + 2K+, again releasing silica and potassium (Ehrig, 2022).
• Intense sericitic (muscovite), grading to advanced argillic alteration occurs mainly at shallow depths to the ENE of the central hematite-quartz-barite breccia zone. Granite-rich breccias are bleached with >35 wt.% sericite accompanied by 5 to 10 wt.% Fe, with <0.3 wt.% Cu, but may contain >0.3 g/t Au. Relict orthoclase occurs, along with ~1.5 wt.% hydrothermal albite, with rare zunyite, kaolinite and diaspore. This alteration is commonly accompanied by strongly altered mafic-ultramafic dykes and intrusions. It also occurs as isolated remnants in hematite-altered breccias in the northwestern extension of the deposit and near the edges of the hematite-quartz-barite breccia (Ehrig et al., 2012). Alteration of muscovite to kaolinite (or pyrophyllite and andalusite, depending on the pH) is represented by the equation: 2KAl2(AlSi3O10)(OH)2 +2H+ + 3H2O → 3Al2Si2O5(OH)4 + 2K+. The subsequent dissolution of kaolinite comprises:
Al2Si2O5(OH)4 + 6H+ → 2SiO2 + 2Al3+ + 5H2O, again releasing silica to the acidic hydrothermal fluid (Ehrig, 2022).
• Hydrothermal quartz was released during alteration of feldspars, muscovite and chlorite (as indicated by the formula above), and occurs locally in some locations as 1 to 5 mm thick microveinlets with apatite, magnetite and/or siderite in significantly altered, but largely un-brecciated granite, and as anhedral to subhedral crystals in breccias. It is uncertain whether these account for all of the silica formed during hydrolytic alteration, and most would appear to have been removed in solution by hydrothermal fluids. It is also found as 20 to 50 m thick quartz veins in altered Roxby Downs Granite in the extreme SE of the Olympic Dam Breccia Complex, where it forms a NNW-SSE elongated, >800 x 200 m zone within altered, weakly brecciated granite. These quartz veins are not geochemically anomalous in any trace metals. Timing of the quartz veining has been difficult to establish. Silicification also occurs as irregular zones along the edges of the hematite-quartz-barite HEMQ breccias (Ehrig et al., 2012).
• Siderite, fluorite and barite alteration occurs as veins and as breccia matrix infill, and is zoned across the deposit. Barite tends to be best developed in the shallow core of the Olympic Dam Breccia Complex in the HMEMQ Breccia, grading out and down to overlapping fluorite which passes outward into siderite. As detailed above, siderite typically occurs at depth and on the edges of the deposit, accompanied by and replacing magnetite, and is less susceptible to replacement by hematite than is magnetite. The strongest fluorite corresponds closely to the highest Cu ores. Pyrite and chalcopyrite can also accompany siderite, along with fluorite, although the latter is more strongly associated with hematite alteration and chalcopyrite, bornite and chalcocite. Barite is closely associated with hematite in hematite-quartz-barite breccias which lack sulphides, and the rocks immediately surrounding those breccias. Elsewhere, barite occurs with fluorite, chalcopyrite, bornite and chalcocite. Siderite and barite do not usually occur together, except in late stage barite-fluorite-siderite veins that cut the Olympic Dam Breccia Complex (Ehrig et al., 2017).
• The bedded clastic facies have, in places, been subjected to intense texturally destructive hematite alteration that overprints the contacts with neighbouring facies and altered Roxby Downs Granite. This alteration includes sericite and chlorite that is replaced by hematite. However, overall the 'bedded clastic facies' have not been brecciated, mineralised or altered to the same degree as the Roxby Downs Granite. Again, apart from specific areas, the green mudstones and sandstone (KASH facies) is little altered, without an IOCG signature, while the red mudstone and sandstone (KHEMQ facies)
has an IOCG chemical signature and is hematite rich, possibly due to pervasive alteration of porous sandstone and its mudstone wallrocks.
• Carbonate minerals - the deposit contains a volumetrically substantial and mineralogically diverse component of carbonate minerals which are always associated with ore sulphides and uraninite. Siderite and siderite-rhodochrosite-magnesite in solid solution are the dominant carbonate types, whilst calcite, dolomite-ankerite solid solution and REE-fluorocarbonates are locally abundant. Isolated carbonate grains are typically compositionally zoned (both simple or oscillatory), whilst replacement textures, including mutual replacement of carbonates by other carbonates and hematite are common (Apukhtina et al., 2020). Dating of Ca-Fe-Mg-Mn-carbonate gangue minerals which are associated with ore minerals reflects virtually every stage of mineralisation in the prolonged, multi-stage history of brecciation and mineralisation from 1.59 to 0.4 Ga. Sm-Nd and Pb-Pb isotope dating of calcite veins in ~1.59 Ga IOCG ore indicate 1.59 to 1.55 Ga deposition ages, whilst the structurally youngest carbonates yield ~0.50 Ga Sm-Nd ages. The latter are unbrecciated fluorite-barite veins and carbonate matrix in polymict conglomerate above the breccia complex, the inferred carbonate ages of which are broadly consistent with radiometric dates for other hydrothermal minerals e.g., hematite, uraninite, apatite, fluorite associated with these veins (Maas et al., 2022).
Carbonates are found in all lithologies at Olympic Dam, occurring in the following associations:
i). Pink to white, un-brecciated, coarse-grained calcite veins in weakly, in situ brecciated Roxby Downs Granite and rhyolite. These veins crosscut texturally early, ~1590 to 1570 Ma magnetite-pyrite-apatite-quartz-uraninite-dominant assemblages and locally include barite, fluorite, quartz, hematite, anhydrite and synchysite, with ~1% sulphides, mainly galena, chalcopyrite and pyrite (Apukhtina et al., 2020). These veins yielded ages of 1546 ±33 Ma (Sm-Nd isochron; Maas et al., 2022) for calcite; and 1585 ±23 Ma (Pb-Pb isochron age; Maas et al., 2022) for calcite and anhydrite, which are broadly consistent with the age of the host granite, and associated apatite (1583 ±7 Ma) and uraninite (1594 ±6 Ma) from the U-rich assemblage they cut (U-Pb ages; Jagodzinski, 2014; Apukhtina et al., 2017).
ii). Carbonates in coarse- to fine-grained, strongly brecciated granite, accounting for ~88% of all carbonates. Siderite is by far the most abundant carbonate, occurring in breccia cement, breccia clasts, breccia-hosted former pores and as carbonate-bearing ooids, in order of decreasing abundance. Multiple generations are evident, varying from almost pure FeCO3 to Mn-Mg-rich siderite which have replaced and overgrown each other, with the end members forming oscillatory zoned carbonates. Siderite-bearing breccia cement carries abundant relicts of earlier minerals, such as magnetite and earlier siderite, that have been partially converted to hematite with local µm U-Mo-W-Sn inclusions, and pyrite with apatite inclusions that has been replaced by chalcopyrite. Siderite is both cogenetic with, and has replaced hematite. Late-stage voids carry barite, quartz, fluorite and chalcopyrite, and rarely galena, sphalerite, Co-Fe-As sulphides including tennantite-tetrahedrite, bornite and chalcocite. They also contain Bi-Ag-Au-Pb-Te-Se alloys, that are commonly found as inclusions in chalcopyrite, as well as U-Th-REE bearing minerals such as monazite, florencite and REE-fluorocarbonates with commonly associated sericite and chlorite. Siderite-rich breccia clasts are up to several cm across, and are typically associated with carbonate vein fragments. They are brown, have strongly variable 'within-clast' grain-sizes, and typically occur within a siderite dominated cement. Siderite within clasts is locally cut by Mg-Mn-rich siderite veinlets and has replaced, or has itself has been replaced by, hematite (Apukhtina et al., 2020). The samples dated by Maas et al. (2022), excluding those suspected of contamination, yielded ages in the range 1.60 to 1.55 Ga (Sm-Nd isotope).
iii). Carbonate veins in 'bedded clastic facies', typically ~1 cm thick, mostly dolomite-ankerite, with µm-scale calcite and Fe-rich carbonate inclusions, in both the KASH and KHEMQ facies. Other gangue minerals in the veins, which are typically internally zoned, include barite, fluorite, quartz, hematite and anhydrite. Anhydrite is restricted to the green sandstone and mudstone facies (KASH). Sulphide minerals within the carbonate veins mirror those of their host bedded facies. Carbonate veins in the sandstone and red mudstone (KHEMQ) also include some tennantite-tetrahedrite (Apukhtina et al., 2020). Sm-Nd isotope data for hydrothermal carbonates yields ages in two fields, the first in the main array between 1.59 and 1.55 Ma, consistent with the age of the host clastics, and a second field at ~ 0.49 Ga (Maas et al., 2022).
iv). Carbonate in mafic and ultramafic igneous rocks, occurring as ~1 cm thick veins of either a). calcite and dolomite-ankerite accompanied by chlorite, sericite and hematite, or b). barite-fluorite. Both vein sub-types also contain quartz, anhydrite, hematite, Ti-oxide and diverse sulphides (Apukhtina et al., 2020). Dolomite-ankerite from picrites were dated at ~1593 Ma; siderite, calcite and dolomite-ankerite from basalts also gave ages of ~1593 Ma; calcite and dolomite-ankerite from Gairdner Dolerite Swarm dykes returned ages of ~825 Ma, while dolomite-ankerite from another generation of basaltic rocks were dated at ~500 Ma (Rb-Sr and Sm-Nd isotopes; Maas et al., 2022).
v). Un-brecciated, massive barite-fluorite dominated veins with minor carbonate which crosscut the Olympic Dam Breccia Complex along NE and east-west arrays. The veins vary from a few mm to ~1 m in thickness, and can be traced for >800 m vertically. They also carry minor Ca-Mg-Fe-Mn- and REE-F-rich carbonates, quartz, botryoidal hematite, chalcopyrite, pyrite, as well as traces of bornite, chalcocite, tennantite-tetrahedrite, safflorite, cobaltite, löllingite, sphalerite, galena, alloclasite, native gold, diverse Bi-Ag-Pb-Se-Te bearing alloys and U-REE minerals, including uraninite, coffinite, brannerite, florencite and REE-fluorocarbonates (Apukhtina et al., 2020). Dolomite-ankerite and siderite from this trend are dated at ~0.5 Ga (Sm-Nd isotopic composition) supported by cogenetic fluorite also with a 0.5 Ga age (Sm-Nd age; Maas et al., 2022).
vi). Intervals largely composed of beige to brown siderite up to several tens of metres thick replacing lithologies within the Masher's Fault zone. This siderite has a wide range of textures, including laminated to drusy, cockade and crustiform. It is un-brecciated and replaces clasts up to several cm across of hematite-dominant 'bedded clastic facies'. In some cases, replacement and concentric overgrowths by fine- to coarse-grained siderite has obliterated all protolith textures. These carbonates are overwhelmingly pure FeCO3, locally replaced by Mg-Mn-rich siderite. The laminated siderites, which are the dominant form, are interpreted to be altered Fe-rich sandstones, overprinted by hydrothermal siderite (Apukhtina et al., 2020). Samples of this association have very coherent Sm-Nd isotope systematics within the Main Array, implying 1.59 to 1.55 Ga ages, broadly supported by the 1598 ±170 Ma Sm-Nd age for 12 data points dated by Maas et al. (2022).
vii). Coarse- to fine-grained carbonate matrix in alternating layers of conglomerate-breccia and sandstone. The beds are immediately above the main hematite-breccia hosted ore deposit, separated from it by the Neoproterozoic unconformity, and are overlain, in turn, by dolomitic-like facies similar to the Neoproterozoic Ediacaran Nuccaleena Formation. The conglomerate-breccia is composed of polymictic, spherical to oval, to slightly angular clasts up to several cm across. Strong hematite alteration and clast-in-clast textures mask identification of the original protolith of most clasts, although Roxby Downs Granite and Gawler Range Volcanics clasts are evident. Other clasts appear to be glacial dropstones. All are set in a sedimentary carbonate matrix. The sandtones are cross-bedded, fine-grained and well-sorted, composed of rounded, ~3 mm diameter quartz-dominated grains, with local hematite-rich layers. The carbonate matrix/cement in both lithologies is predominantly strongly zoned dolomite-ankerite grains (Fe-poor cores → Fe-rich rims) with associated, intergrown barite and celestite. Pyrite, chalcopyrite, bornite and minor altaite are locally disseminated throughout both the conglomerate clasts and cement, whilst sulphide-carbonate veins are commonly restricted to individual clasts. Immediately above the unconformity, the dolomitic facies are thinly bedded, fine-grained (~50 µm) with quartz and possibly granite derived accessories, including apatite, zircon and Ti-oxides. There is evidence that dolomite in this dolomitic facies has undergone post depositional Fe alteration, e.g., ankerite rims on dolomite crystals and ankerite veins crosscutting dolomite, as well as crosscutting barite-carbonate veins (Apukhtina et al., 2020). The Sm-Nd isotope signatures of these carbonates, with their characteristic high and variable Sm/Nd, clearly yield an Sm-Nd age of ~0.5 Ga (Maas et al., 2022). If the ~1590 Ma deposit has been upgraded by an ~0.5 Ga unconformity-style uranium mineralisation event, this alteration association would represent the fluid interface.
The ages of these carbonates, all of which have varying degrees of associated 'ore sulphides' and uraninite, are interpreted to reflect three periods in which mineralisation was deposited or re-deposited, namely ~1.60 to 1.55 Ga, ~0.82 and ~0.5 Ga. The dates are consistent with ages measured for other minerals, both gangue and ore, providing further evidence for a prolonged multi-stage history of ore formation and reinforcement of grade and tonnage through repeated overprints (Maas et al., 2022).
MINERALISATION
There is a strong enrichment of a broad range of elements that have a direct positive correlation with Fe, for below and up to ~45 wt.% Fe (or ~75 wt.% hematite). These elements include Cu, U, Au, Ag, F, S, C, As, Ba, Bi, Cd, Co, Cr, Fe, In, Mo, Nb, Ni, P, Pb, Sb, Se, Sn, Sr, Te, V, W, Y, Zn and REE. All of these are regarded as being primarily of hydrothermal origin. In particular, there is a strong spatial correlation between Cu, U, Au and Ag. Above ~75 wt.% hematite, the concentrations of Cu, U3O8, and Au sharply decrease (Ehrig, McPhie and Kamenetsky, 2012), whilst below ~30 wt.% hematite, there is also a marked decrease. These trends are much more extreme for chalcocite, decreasing through bornite to chalcopyrite, with little correlation between Fe and Cu grade in pyrite(-chalcopyrite) mineralisation. In contrast, elements interpreted to be affiliated with granite, such as Al, Be, Ca, Hf, K, Li, Mg, Mn, Na, Rb, Si, Th, Ti and Zr are negatively correlated with Fe, with the degree of depletion increasing proportional to the Fe concentration (Ehrig, McPhie and Kamenetsky, 2012).
The hematite content is proportional to the increase in permeability generated by brecciation towards the spatial centre of the deposit (Reeve et al., 1990), with sulphides being precipitated within the breccia matrix as the volume of matrix increased (Ehrig, McPhie and Kamenetsky, 2012). Clark and Ehrig (2019) conclude therefore, that between ~30 and 75 wt.% hematite, the permeability is optimal for maximum sulphide precipitation, if the hydrothermal fluid-rock interaction is sufficiently high. Above 75 wt.%, the permeability becomes significantly occluded, reducing fluid flow and the ability to precipitate economic concentrations of sulphides.
Ca and Mn were initially depleted through amphibole and biotite being altered to chlorite, whilst the early sericite alteration of sodic plagioclase depleted Na. This was followed by enrichment of F and Mn via the precipitation of fluorite and Mn-bearing siderite when Cu mineralisation reached ≥1000 ppm.
In Cu-barren and weakly to moderately mineralised levels of ≤3000 ppm Cu, the Olympic Dam deposit is enriched in LREE (light rare earth elements), whereas the MREE (medium rare earth elements) and HREE (heavy rare earth elements) are depleted. The MREE and HREE become enriched in mineralisation of ≥3000 ppm Cu. The REE pattern for samples with >50% Fe and ≥3000 ppm Cu are depleted relative to samples with <50% Fe (Ehrig et al., 2012).
Dmitrijeva et al. (2019) studied the lithogeochemical zoning of the Olympic Dam deposit, and found the signature of the deposit was composed of two geochemical associations, which exhibit distinct, but spatially overlapping distributions: i). The first group, Cu-U3O8-Se-S, which comprises the main economic and closely associated elements, has a concentric zonation, outward and downward from a Cu-barren, high-Fe, hematite-quartz-barite-bearing breccia (HEMQ) in the upper central core of the deposit; this signature is also found in many of the altered mafic-ultramafic dykes. ii). The second group, Au-W-Mo-Sb-As, forms a column with a vertical extent of >1800 m, still open at depth, and is located in the southeastern lobe of the deposit where it spatially coincides with >5% Fe hematite and volcanic breccias within the Olympic Dam Breccia Complex. This second signature is especially evident at depths below 1200 m, as well as in generally shallow, hematite-quartz-barite bearing breccias (HEMQ) and hematite-quartz sediments (KHEMQ) of the 'bedded clastic facies', but not the chlorite-bearing more mafic facies sediments (Dmitrijeva et al., 2019). The Au-W-Mo-As-Sb signature is overwhelmingly related to the occurrence of compositionally-zoned hematite, which hosts most of the W, Mo and Sn throughout the deposit, as well as a significant part of the U (Verdugo-Ihl et al., 2017), and to sulphides hosting Sb and As, which include pyrite (As); tetrahedrite-tennantite (Sb-As); and cobaltite (As) (Ehrig et al., 2012). Gold is mostly native, alloyed with Ag (and rarely Cu), or as Au(±Ag)-tellurides, occurring as inclusions in sulphides and hematite. This vertical mineralised column is considered likely to be related to the intersection between the major subvertical WSW-ENE and NNW-SSE striking fault systems which acted as a hydrothermal-fluid conduit. However, the concentration and zoning of the first group signature, while being controlled by the same mineralisation/alteration corridor, appears to be spatially independent of the Au-W-Mo-As-Sb association, i.e., two separate mineral associations taking advantage of the same conduit.
Ehrig et al. (2017) regard the principal controls of Cu grade within the deposit, and by inference, U, Au and Ag, in decreasing order of significance, to be: i). sulphide species and abundance; ii). intensity and type of iron oxide alteration, i.e., hematite or magnetite; iii). structure and geometry; and iv). protolith lithology.
Ehrig and Clarke (2020) demonstrated by plotting the very high grade intervals (i.e., >5% Cu, >2.5 g/t Au), that such high grades closely follow faults, particularly fault intersections, and are closely associated within and around the very reactive ~1590 Ma mafic to ultramafic dykes, the distribution of which are controlled by the same faults. The very high grade Cu is particularly evident in the core of the NW neck of the resource, whilst gold is more heavily concentrated in the core of the deposit.
The overall paragenetic sequence of sulphide precipitation within the deposit is sphalerite → galena → pyrite → chalcopyrite → bornite → chalcocite, although sphalerite and galena are only found in trace amounts. However, locally, sphalerite contents may be up to 2 wt.% in distal breccia (Ehrig et al., 2012), and late-stage veinlets of galena occur within the HEMQ hematite-quartz-barite breccia of the barren core (Corriveau et al., 2022).
The occurrence of each of the main economic metals can be summarised as follows:
• Copper - The dominant copper sulphide minerals within the deposit are chalcocite/digenite/djurleite (collectively referred to as chalcocite), bornite, chalcopyrite and pyrite, which occur in the relative proportion of 25, 50, 18, 7%, respectively, with lesser sphalerite, galena, molybdenite, tennantite-tetrahedrite and traces of native copper. These sulphides predominantly occur as disseminated grains in both the breccia matrix and clasts, with grain sizes that vary from <20 µm to several millimetres, averaging ~100 µm. Sulphides may also, but rarely, occur as veins in granite-dominated breccias.
Tennanite-tetrahedrite and enargite occur in relatively small amounts and appear to be associated with ores in the deep mineralised zone, above and surrounding mineralisation below –950 mRL. Covellite, native copper and tenorite/cuprite are very rare. Most sulphides do not display textures indicative of supergene formation, suggesting they are hypogene in origin. However, covellite, which occurs along the edges of and in cracks within bornite grains, at or near the unconformity with the overlying Neoproterozoic cover sequence, does suggest it is related to a supergene influence. Never-the-less, if a significant supergene blanket was developed, it has since been removed by Meso- to early Neoproterozoic erosion. Native copper and tenorite/cuprite are locally found in association with chalcocite, but usually not near the overlying unconformity. Pyrite, chalcopyrite, bornite and chalcocite may be intergrown with the major gangue minerals of hematite, quartz, sericite, orthoclase, chlorite, barite, fluorite and siderite, whilst bornite and chalcocite are only rarely associated with siderite.
There is a distinct upwards and inwards zonation of sulphide/copper species within the deposit, from: pyrite → chalcopyrite-pyrite → chalcopyrite as exsolution lamellae in brown bornite → purple bornite-chalcocite symplectites → digenite lamellae in chalcocite → occasional native copper (Reeve et al., 1990; Ehrig, McPhie and Kamenetsky, 2012; Ciobanu, Cook and Ehrig, 2017).
Two generation of pyrite have been recognised, namely: i). coarse-grained euhedral crystals that are commonly associated with magnetite, with or without chalcopyrite, although the chalcopyrite is not seen to replace the pyrite; and ii). a more common anhedral to subrounded species that is partially replaced by chalcopyrite. Pyrite replacement of chalcopyrite has not been observed. Very rare small pyrite grains have been found in massive bornite.
Chalcopyrite may occur as i). isolated grains; ii). co-precipitations with bornite: iii). grains being replaced by bornite; or iv). rare replacement of bornite.
Bornite occurs as i). isolated grains; ii). grains being replaced by chalcocite, or iii). most commonly, as myrmekitic bornite-chalcocite intergrowths.
Chalcocite occurs as isolated grains and rarely replaces bornite.
Chalcopyrite-bornite pairs are not as common as chalcopyrite-pyrite or bornite-chalcocite. The pairs chalcopyrite-bornite, bornite-chalcocite and chalcocite-digenite may represent the progressive addition of Cu at temperatures of >300°C, and form an upward and inward gradient of increasing Cu:Fe and decreasing S:Cu+Fe ratios (Ciobanu, Cook and Ehrig, 2017). However, as the transition from chalcopyrite to bornite-chalcocite in the Olympic Dam deposit invariably coincides with a general 2 to 4 times increase in Cu grade, the actual amount of sulphur present remains comparable throughout (e.g., 1% Cu as chalcopyrite = ~1% S, whilst 2.5% Cu as bornite = ~1% S; and 4% Cu as chalcocite = ~1% S). It should be noted most o the higher tenor chalcocite and bornite were apparently formed at lower temperatures of 250 to 150°C.
Other base metals are found on the margins of the Cu-U-Au-Ag deposit, where chalcopyrite-sphalerite-galena can be co-genetic or sphalerite-galena may be later than chalcopyrite. A galena-chalcopyrite association is regarded as cogenetic with pyrite, although the galena is generally non-radiogenic, implying it formed early, prior to the system becoming enriched in radiogenic lead.
Ehrig, McPhie and Kamenetsky (2012) describe an outer, distal and deep or paragenetically early Mo-W-Sn-As-Sb enrichment at geochemically anomalous levels which may be overprinted by Cu-Pb-Zn, in the south-eastern lobe of the deposit defining a further sulphide zonation. This zone is postulated to grade inwards and upward → (Cu-Pb-Zn) → and the barren (hematite-quartz-barite) breccia upper core to the deposit (Corriveau et al., 2022). Deep drilling has intersected this Mo-W-Sn-As-Sb signature overprinted by grades of between >0.5 to 4% Cu at depths of from ~900 to 1800 m below surface (Fig. 4, section G-H). The Mo-W-Sn-As-Sb signature is geochemically distinct and in the deep section is bounded by faults. It is most likely related to the Au-W-Mo-Sb-As signature of Dmitrijeva et al. (2019). The host hematite breccia contains recognisable clasts of KHEMQ, red sandstone-mudstone of the 'bedded clastic facies', but also appears to overlap the VBx volcanic breccia. It is also intruded by ~1950 Ma mafic to ultramafic dykes. The adjacent fault block, between the Woodall and Woodall Fault Junior is characterised by K feldspar alteration and contains veins of quartz-tourmaline, quartz-carbonate and quartz. This high grade zone is interpreted to lie within a fault slab of 'bedded clastics facies' that has been tilted and collapsed into the Olympic Dam Breccia Complex during the life of that complex's formation (After Ehrig, 2022 SAEMIC conference). As such it may reflect an initial interaction with hydrothermal fluids above the main breccia complex prior to collapse, and subsequent alteration and mineralisation within the breccia complex. It appears to lie within the transition from the chalcocite-bornite to bornite-chalcopyrite zones.
• Uranium is predominantly present as fine-grained particles of uraninite [(U,Pb,Ca,Y,REE)O2], coffinite [U(SiO4)1-x(OH)4x] and brannerite [(U,Ca,Y,Ce)(Ti,Fe)2O6], disseminated throughout, interstitial to the sulphides and gangue minerals, and accounting for >80% of the uranium in the deposit (Ehrig, et al., 2021). The grain size of the uranium minerals ranges from <0.5 to >1000 µm, averaging ~20 µm, with uraninite-coffinite and coffinite-brannerite commonly complexly intergrown. Coffinite and brannerite are interpreted to have formed as a result of the alteration of uraninite. The uraninite occurs as fine <30 µm euhedral grains that are primary, and as anhedral to subhedral to round grains, which are <30 to ~100 µm in diameter and are chemically and texturally distinct from the euhedral form. The anhedral to subhedral/rounded grains, which are prominent in high-grade ore zones, form: i). larger massive aggregates; and rarely, ii). microveinlets that can be as much as 1 mm wide (Macmillan et al., 2016, 2017).
Another ~15% of the uranium occurs as submicron-sized particles and lattice substitutions in hematite, along with elevated Mo-W-Sn (Ciobanu et al., 2014), whilst <5% occur in sulphides, including pyrite, chalcopyrite, bornite and chalcocite, as well as in uranothorite, thorianite, the REE minerals bastnäsite, florencite and synchysite, and in xenotime, monazite, zircon and the crandallite group. Uraninite has a slightly stronger association with hematite, whilst coffinite and brannerite are a little more commonly associated with non-hematite gangue (Ehrig, et al., 2012, 2017).
Deposit-wide, the average relative proportions of brannerite, coffinite and uraninite are 31, 56 and 13% respectively, although the latter increases to >40% at high Fe concentrations. The uranium minerals have no statistically significant preferential association with pyrite, chalcopyrite, bornite or chalcocite. Unlike the Cu ±Fe sulphides, the uranium minerals do not form systematically zoned patterns across the deposit (Ehrig, et al., 2017). The uranium mineralisation was deposited and remobilised during multiple cycles of dissolution and re-precipitation of these uranium ore minerals, and the composition of each of these minerals is complex and very variable (Ehrig and Clark, 2020)
The high abundance of uranium in the Olympic Dam deposit allows U-Pb dating of ore minerals. As detailed above, two types of uranium mineralisation are evident, namely i). fine euhedral primary grains of uraninite, and ii). anhedral to subhedral/rounded grains of uraninite, coffinite and brannerite. U-Pb dating of the fine-grained euhedral uraninite yields ages of ~1.59 Ga (Macmillan et al., 2016; Apukhtina et al., 2017). These euhedral uraninite grains contain high total rare earth element (REE) levels, have relatively unfractionated REE patterns with low Ce/Lu, pronounced La and Eu depletion and low Y/Ho ratios. In contrast, the texturally distinct anhedral to subhedral/round uraninite grains have variably preserved U-Pb ages of ~0.5 Ga, and are characterised by lower total REE levels, and by REE patterns with very low La/Sm ratios, pronounced peaks of Sm, and an absence of Eu anomalies (Ehrig et al., 2021). Apart from these younger age dates, there is a pronounced deficit of Pb present compared to that expected in a U deposit formed at 1.59 Ga. This is best accounted for by at least part of the U having been added as late as 1 billion years subsequently. Uranium mineralisation of this younger generation is locally found with partially preserved, yet significantly altered remnants of the older ~1.59 Ga euhedral uraninite (Macmillan et al., 2016), and dominates the highest U ore grades in the deposit, also consistent with the younger U-Pb ages. Ehrig et al. (2021) note that the REE patterns in this younger uraninite generation resemble REE signatures typical of low-temperature uraninite in the large, high-grade Proterozoic unconformity-related U deposits of Canada and northern Australia (Fryer and Taylor, 1987; Frimmel et al., 2014).
Additional evidence for a major Late Neoproterozoic to Cambrian U mineralisation event is provided by the Pb isotope composition of thousands of hydrothermal chalcopyrite, bornite and chalcocite grains analysed at Olympic Dam. These data define a 207Pb/206Pb ratio in the range 0.07 to 0.06. U concentrations
in these sulphides, including those with highly radiogenic Pb, vary widely, but the vast majority have low U/Pb ratios, interpreted to imply the radiogenic Pb did not evolve within the low-U sulphide carrier minerals, but was inherited from associated U minerals. The timing of the production and release of highly radiogenic Pb from U minerals, and its subsequent capture by Cu sulphides, can be calculated to have occurred between 0.9 and 0.6 Ga, if Pb release occurred in the recent geological past. If Pb release occurred earlier, the parental U minerals cannot be older than ~0.7 Ga. Ehrig et al. (2021) conclude the 'remarkably' homogeneous and low 207Pb/206Pb in the Cu sulphide minerals implies a major period of U mineral formation at 0.7 to 0.5 Ga, broadly consistent with the ~0.5 Ga ages of non-euhedral uraninite. The presence of this young radiogenic Pb in the Cu sulphides is also interpreted to imply widespread concomitant modification of precursor, and possibly growth of new, sulphide minerals.
In addition to these two generations of uranium mineralisation, age dating of uranium minerals also suggests the possible addition of minor U during the ~1200 to 1160 Ma Musgravian Orogeny (Ehrig, 2019)
Ehrig et al. (2021) note that the Olympic Dam Breccia Complex is truncated by the Neoproterozoic Marinoan glaciation event during the Cryogenian, and unconformably overlain by the cover sequence deposited under periglacial conditions during a period that coincided with a rise in atmospheric oxygen (Lyons et al., 2014). As such, they suggest, the younger, 0.7 to 0.5 Ga U mineralisation may have been related to circulation of oxidised basinal fluids from above the unconformity which enhanced the solubility and mobility of the pre-existing 1.59 Ga uranium mineralisation.
Age dating of uranium minerals also suggests the possible addition of minor U during the ~1200 to 1160 Ma Musgravian Orogeny (Ehrig, 2019).
• Gold occurs occurs as Au-Ag-Cu-Fe electrum, calaverite, petzite and tetra-auricupride [AuCu] as i). inclusions within, or attached to, sulphide minerals; ii). inclusions in quartz, hematite and barite in gold-dominant, sulphide free zones on the leading edges of barren hematite-quartz-barite HEMQ breccias, juxtaposed against Cu sulphide mineralisation in the adjacent hematite breccias; and iii). inclusions in sericite and/or quartz in gold-dominant zones in intensely muscovite and paragonite altered granite breccias (Ehrig, et al., 2012, 2017).
Gold particles are typically ~1 to 20 µm across, although bonanza veins and rare large nuggets are found in hematite-rich gold-only zones (Ehrig, McPhie and Kamenetsky, 2012). Two distinct electrum populations have been recognised: i). >95% Au with <5% Ag+Cu where associated with sulphides, and ii). <90% Au with ~10% Ag+Cu+Fe where associated with sericite, quartz, hematite or barite, in the absence of sulphides (Ehrig, et al., 2012, 2017).
• Silver is predominantly found as >10 µm hessite micro-inclusions in bornite, but also occurs in electrum, petzite, acanthite, allargentum and rarely as native silver. Bornite, chalcocite and tennantite-tetrahedrite may also contain sub-micron inclusions of native silver.
• Rare Earth Elements - the principal REE and their grades at Olympic Dam are ~0.17 wt.% La and 0.25 wt.% Ce, contained in bastnäsite and florencite, with lesser synchysite, monazite, xenotime, zircon and crandallite. These minerals occur as grains disseminated between both gangue and sulphide minerals, with an average grain size of 35 µm. REE also occur in minor to trace concentrations in uranium minerals. However, whilst Olympic Dam ores contain anomalous levels of REE, the concentrations are not sufficiently high to justify economic extraction at present (2022).
The estimated indicative REE composition of the Olympic Dam deposit are as follows (Ehrig, K., AusIMM Geoscience Society Webinar: Olympic Dam, the world's largest IOCG deposit - But what about rare earth elements?, 28 July, 2023):
Rare Earth Elements in ppm = La - 1000; Ce - 1515; Pr - 129.7; Nd - 343.9; Sm - 34.11; Eu - 10.62; Gd - 20.29; Tb - 2.55; Dy - 13.76; Ho - 2.68; Er - 7.74; Tm - 1.13; Yb - 7.47; Lu - 1.03; Y - 73.51. LREE - 3053; HREE - 110; TREE - 3163.
Rare Earth Oxides + Y in ppm = La - 1173; Ce - 1774; Pr - 151.8; Nd - 401.1; Sm - 39.55; Eu - 12.30; Gd - 23.38; Tb - 2.94; Dy - 15.79; Ho - 3.07; Er - 8.85; Tm - 1.30; Yb - 8.50; Lu - 1.18; Y - 93.35. LREO+Y - 3575; HREO+Y - 135; TREO+Y - 3710.
Within a total mineral resource of 11.68 Gt of ore (BHP Annual Report, 2022), this equates to 43.333 Mt of contained TREO+Y. However, Ehrig (2023) points out, these grades are in excess of one order of magnitude less than grades reported by Wang et al (2015) for mines in production, with the exception of alluvial and ion-absorption mineralisation.
The REE minerals in the olympic Dam deposit are typically <9 µm in size, and after grinding to 80% passing -75 µm, the REE minerals remain significantly locked within gangue minerals.
Mineralised alkaline ultramafic to mafic dykes - as detailed above in the Geology section, alkaline ultramafic to mafic dykes, lavas and possibly sills comprise ~30 vol.% of the Olympic Dam Breccia Complex, including those interpreted as the protoliths of heavily hematite altered rocks (Ehrig, 2022, from unpublished mass balance calculations). Johnson and McCulloch, (1995) estimated the ultramafic to mafic rocks accounted for >13 vol.% of the breccia complex, but contained ~50% of the copper in that complex. By their nature, the protoliths of these mafic/ultramafic rocks are highly chemically reactive and typically contain chalcocite-bornite dominant sulphide assemblage, irrespective of where they intrude the broader zonation pattern. Mineralisation may be hosted within the mafic protoliths, generally accompanied by brecciation and strong hematite alteration (Figs. 2 and 3) and, or be concentrated along the margins of dykes that are otherwise barren. Some of the highest copper and gold grades (i.e., >4 wt.% Cu and >2 g/t Au) across the deposit are hosted in intensely hematite altered mafic/ultramafic dyke protoliths (Clark and Ehrig, 2019).
Shallow epithermal mineralisation has been encountered nearer surface and distal to the Olympic Dam Breccia Complex. Examples illustrated in Corriveau et al. (2022) include hematite-fluorite-sulphide 'pisoliths' supported by a hydrothermal siderite cement, interpreted to represent sub-surface hydrothermal boiling textures whereby vugs are infilled from the outside, inward. Another example is of chaotic low-temperature 'epithermal' quartz veins found at a level interpreted to represent a near-surface setting. These are interpreted to represent precipitation resulting in the pressure-temperature reduction and consequent boiling of fluid that escaped from the early overpressured hydrothermal system responsible for the Olympic Dam Breccia Complex.
Two ore types have been delineated within the Olympic Dam Breccia Complex (Ehrig and Clark, 2020), namely a:
• bulk tonnage, low grade, >0.6% Cu equivalent, disseminated chalcopyrite(-pyrite) body of ~10 Gt of ore, represented by the Open Pit Resource as listed in the BHP 30 June 2022 published resources report (see below). This mineralisation is reasonably homogenous and spread throughout the complex, with a slight increase in grade towards the centre of the deposit; and a
• lower tonnage, high grade, >2% Cu equivalent, occurring as a series of structurally focussed, discrete ore shoots that have a strongly anisotropic and variable geometry, as well as a restricted, but similarly anisotropic halo of ~1% Cu disseminated sulphides. This mineralisation is hosted in the fluorite-rich hematite breccias containing bornite-chalcocite, with lesser chalcopyrite. The ore shoots are controlled by: i). zones of intense brecciation accompanied by a high rate of fluid-rock interaction; ii). chemical dissolution > physical brecciation, and iii). local dilatant zones developed at structural intersections.
Satellite Mineralisation
A distal body of satellite mineralisation has been outlined ~4 km NE of the main Olympic Dam deposit. It has dimensions of ~2 x 0.5 km in plan, and 800 m in vertical extent, and lies on a broadly coincident gravity and magnetic geophysical anomaly down-strike from the regional scale Jubilee Fault. The alteration mineralogy and geochemical signature are similar to that seen at the margins of the Olympic Dam Breccia Complex. Alteration comprises hematite-muscovite imposed on the Roxby Downs Granite, visible as red-stained K feldspar that is associated with sparse copper-(iron)-sulphide mineralisation that rarely exceeds 1 wt.% Cu (Kontonikas-Charos et al., 2017). Relict magnetite-apatite-quartz alteration is overprinted by hematite-sericite alteration, although a broad magnetic susceptibility anomaly is evident throughout the satellite mineralised zone, likely reflecting deeper, peripheral alteration linked to the broader Olympic Dam mineral system (Corriveau et al., 2022).
GEOPHYSICAL EXPRESSION
Geophysical data, including deep seismic, magnetotelluric (MT), gravity and magnetic, reflect the 3D setting of the Olympic Dam deposit from a crustal to deposit scale.
Continental-scale seismic tomography datasets indicate the boundary between the lithospheric mantle and underlying asthenosphere is thickest at 180 to 220 km to the west of Olympic Dam, beneath the central Gawler Craton (Kennett et al., 2013), where the depth of the Moho is ~54 km. The latter shallows towards the north, related to offsets across north-dipping trans-crustal faults. To the east, immediately to the SW of the Olympic Dam deposit, the Moho is at depth of ~39 km and becomes slightly deeper to the NE where it is imaged at ~42 km (Drummond et al., 2006). Seismic reflection data shows an offset of the Moho beneath Olympic Dam with slightly thicker crust to the north of the deposit, interpreted to reflect a large crustal-scale fault zone.
Two- and three-dimensional magnetotelluric (MT) modelling to a depth of 60 km has been undertaken on data obtained from traverses across and along the eastern margin of the Gawler Craton, including the Olympic IOCG Province. This modelling revealed a number of domains, as follows (after Heinson et al., 2018):
• A conductive surface layer, corresponding to Neoproterozoic cover sequence of the Stuart Shelf, overlain by transported Tertiary sediments. This layer is similarly evident in reflection seismic sections. The cover thickness around the Olympic Dam deposit is shown to be ~300 m, which is consistent with drilling evidence, but thickens significantly into a deep-rifted basin to the NE, with sedimentary thickness of >2 km.
• Archean to Palaeoproterozoic Gawler Craton crust to the SW is characterised by high resistivity, >1000 Ωm values in MT data, and by high seismic reflectivity, to a depth of >60 km.
• The Palaeo- to Mesoproterozoic crust to the NE has similar high resistivity characteristics to that of the Archaean detailed above, and dips to the NE.
• Between the two high resistivity (low conductivity) domains of the previous two points, there is a 50 km wide zone of low-resistivity, <10 Ωm, that is imaged along the margin of the Archaean Gawler Craton at a depth extending from 15 to 40 km in the mid to lower crust. This highly conductive lower crustal zone is clearly evident in both the long-period MT responses and vertical field anomalies. This conductor is closely coincident with a region of low seismic reflectance, and is centred directly beneath the deep-rifted Neoproterozoic basin to the north east of Olympic Dam. This suggests a zone of crustal rheological weakness. The low seismic reflectance defines a zone where the pattern of reflectors seen to the SW, NE and at shallower levels, fades into a bland area lacking well defined texture. The least resistive, ~1 Ωm part of this domain (in MT data), has an upper termination at ~15 km depth which corresponds to the brittle-ductile transition. Further interpretation of the data, including stations to the east and west of the major NNE-SSW traverse, showed that this major conductivity anomaly was strongly developed at a depth of 25 km, but did not persist to 50 km below the surface. This 3D model showed Olympic dam on the SW gradient of the conductivity high, which is centred to the NE. However, an image that included deeper data in Reid (2019) shows this major conductivity high reappearing to depths of ~100 to 150 km, below the Moho, where it is offset to the east. The shallower and deep conductivity highs are linked by a saddle of marginally lower conductivity. The Moho also appears to be offset by ~3 km, NE-side down, across this composite conductivity high domain, suggestive of a large transcrustal scale fault zone (Reid, 2019). This fault would appear to confirm the similar interpretation of seismic tomography mentioned above. In plan, the resistivity model at an upper lithospheric mantle depth of 55 km, derived from AusLAMP magnetotelluric data (Thiel et al., 2016), shows this deep conductivity high to underlie the Olympic IOCG Province where it is strongly developed to the NE of Olympic Dam, extending to below Prominent Hill and Cairn Hill in the NW. To the south, it decreases in intensity, but then redevelops over a narrower width to continue below Carrapateena (Reid, 2019).
• Three narrow, enhanced conductivity/low-resistivity, ~100 Ωm 'fingers' branch from the top of the major conductive domain at the brittle-ductile transition depth of ~15 km, and extend to the base of the sedimentary cover layer detailed in the first point above. Heinson et al. (2018) state these low-resistivity branches "align remarkably with the spatial location of the major IOCG-U mineral deposits"/occurrences at Wirrda Well, Olympic Dam and Vulcan that are adjacent to the MT transect. The most intense is that below Olympic Dam. These same features are also apparent in processed seismic section as distinct breaks in reflectance horizons and destruction of seismic texture, with an overall low reflectivity. The same authors note however, that these low-resistivity features are not spatially aligned with significant mapped crustal faults.
This pattern of resistivity lows and bland seismic data described in the last two points appears to overprint the Archaean basement and Palaeo- to Mesoproterozoic rocks of the crust up to and including the Hiltaba suite, but are overprinted by the response from the Meso- to Neoproterozoic cover sequence. They are therefore assumed to be related to the early Mesoproterozoic magmatic/hydrothermal event that affected the eastern Gawler Craton, related to channeling of magmas and/or associated metasomatic fluids into the middle and upper crust. Wise and Thiel (2020) suggest this conclusion is supported by the observation that the lower crustal conductors can be traced to surface expressions of magmatic suites such as the A-type Hiltaba granites and Gawler Range Volcanics in the Olympic Domain. It has been suggested that the high Fe content as magnetite in these A-type granites may in part explain elevated conductivities (Yang and Emerson, 1997). However, anomalous fluorine enrichment is regionally associated with these granites and accompanying alteration minerals, and is also a characteristic of the Olympic Dam deposit (McPhie et al., 2011; Xing et al., 2019). Xing et al. (2019) discussed the potential role of fluorine as a mobilising agent, in the form of the highly corrosive hydrofluoric acid, to dissolve and transport U and REE, as well as increasing silica solubility, thus promoting porosity and enhancing fluid pathway connectivity on both a crustal and deposit scale. Skirrow et al. (2018) have suggested the deep, below-Moho zone of high conductivity and bland seismic character, may reflect the presence of a peridotite mantle that has undergone earlier subduction related metasomatism, and been altered to hydrated olivine (e.g., clinohumite), garnet and phlogopite (which may bear fluorine). Experimental studies by Li et al. (2017) have endorsed fluorine as a mechanism for producing high conductivities. Wise and Thiel (2020) conclude that F/Fe-rich magmatic/hydrothermal fluid flow and consequent alteration, would therefore also explain the apparent destruction of seismically reflective lower crust and any associated hydrothermal alteration could leave behind minor mineral phases with enhanced conductivity, e.g. sulphides and F-rich silicates such as phlogopite.
It is therefore possible that the deep, highly conductive domains and shallower, more restricted branches in the Olympic IOCG Province may reflect pathway of fluids and magmas responsible for the large scale transfer of iron oxides, Cu, Au and fluorine from reworking and partial melting of a fertilised lithospheric mantle to the upper crust.
On a deposit to district scale, the geophysical expression of the Olympic Dam deposit comprises a 17 mGal gravity anomaly that is closely coincident with an ~1600 nT aeromagnetic anomaly (Rutter and Esdale, 1985; Esdale et al., 2003) that also closely coincides with the outline of the resource. The bulk dry density of the Roxby Downs Granite and lithofacies within the Olympic Dam Breccia Complex has been shown to steadily increases from 2.60 g/cm3 in the outer 'unaltered' granite, inward towards the centre of the deposit, where it is >4.50 g/cm3. The magnetic susceptibility is directly correlated with the magmatic and hydrothermal magnetite content of the host rock and mineralisation, which varies significantly across the deposit. The major rock units have magnetic susceptibilities as follows: the relatively unaltered Roxby Downs Granite ~25 to 50 SI units; altered and weakly brecciated Roxby Downs Granite <1 SI units; Gairdner dykes 100 to 300 SI units; typical hematite-rich breccias ~0.5 to 55 SI units); magnetite-rich breccias >100 SI units; and
barren hematite-rich breccias <1 x 10-3 SI units.
EVOLUTION and DISCUSSION
This section discusses an interpretation of the evolution of the Olympic Dam deposit, predominantly as an initial multistage event straddling 1590 Ma, possibly partially reactivated 100 m.y. later, with further additions and modifications distributed over the following more than 1 billion years, as it was influenced by tectonic events and related fluid circulation regimes.
Summary
The following is an overview of the proposed evolution of the Olympic Dam iron oxide copper-gold-silver-uranium deposit that is discussed in more detail below, including references to sources. The deposit, which was formed at ~1590 Ma in the early Mesoproterozoic, is hosted by the ~1593 to 1592 Ma Hiltaba Suite Roxby Downs Granite and co-magmatic Gawler Range Volcanics, both of which were cooled and solidified prior to mineralisation. The Olympic Dam deposit is interpreted to be the result of the interaction of a number of fluids that originated at different levels, from the lithospheric mantle to the shallow crust. The principal fluid was initiated by the partial remelting of lower lithospheric mantle that had previously been fertilised by subduction during the ~1850 Ma Cornian and 1730 to 1690 Ma Kimban orogenies which had also produced the extensive Donington Suite granitoids. This partial melting is interpreted to be due to a mantle plume, or delamination and detachment of the lower lithospheric mantle. It produced mafic to ultramafic magmas and fluids, the latter in pressure solution with the former, which rose to be ponded as a large magma chamber at a level of neutral buoyancy in the lower crust. Fractional crystallisation of the mafic/ultramafic magmas was the source of the mafic facies of the Hiltaba Suite/Gawler Range Volcanics; while the oxidised felsic/silicic magmas were produced by partial melting of lower crust by the ascending mafic-ultramafic magmas and the underlying plume, and in part by mixing with fractional crystallised products of the mafic magmas. The apex of the major mafic/ultramafic magma chamber was at a depth of ~15 km, near the brittle-ductile transition. Segregation within the chamber led to the accumulation of fluids and magmas as a reservoir in its apex. Both the ponded fluids and magmas were saturated with volatiles, iron, Cu, U, Au, Ag and REE, but limited sulphur. This reservoir was tapped by the major transcrustal ENE-WSW Jubilee Fault, which focussed both metal and volatile, saturated fluid (Fluid A) and mafic to ultramafic magmas along an intersection with a prominent NNW-SSE structure. The reactive metal and fluid saturated mafic to ultramafic magmas, which are estimated to occupy ~30% of the Olympic Dam Breccia Complex, released and precipitated Cu sulphides internally and, when reacting with fluids, on their margins. Fluid A, which was confined and overpressured, inflated and expanded faults and related stress-field initiated fractures at the structural intersection to facilitate ingress of the strongly corrosive fluorine rich fluid to produce a replacive breccia. This breccia, the Roxby Downs Breccia Complex, was developed between 1 and 2 km below the surface, and relatively passively stoped up- and outward in the freshly cooled, brittle, Roxby Downs Granite and Gawler Range Volcanics. In doing so, the hot Fluid A cooled sufficiently to form early albite-actinolite ±magnetite alteration, overprinted by a biotite-magnetite-K feldspar IOA style assemblage at temperaures of >400 to 600°C; then magnetite-biotite with low grade 0.1 to 0.3% Cu pyrite-chalcopyrite at ~450 to 350°C. This temperature range was sufficiently low to increase the Cu-saturation of Fluid A and release enough Cu (and other metals) to combine with the limited sulphur in the fluid, but retained significant excess Cu in solution in the Fe2+ oxidised, hot fluid. This was stage 1 mineralisation, which was probably stimulated by periodic relief of overpressure afforded by movement on the associated fault system. However, the breccia complex then breached the Gawler Range Volcanics into the 'bedded clastic facies'. This resulted in fluid connectivity to be established between Fluid A and the very much cooler, oxidised and sulphate rich Fluid B resident in the continental red-bed OD Basin. This led to the loss of overpressure and produced a hybrid Fluid A-B that had two affects: i). addition of sulphate; and ii). cooling of the combined fluid from the hot Fe2+ magnetite-stable conditions of Fluid A, to a cooler, heavier, Fe3+ hematite stable, but still acid and volatile rich Fluid A-B. While the reduction in temperature reduced the solubility of Cu, this was compensated by the increase in the degree oxidation, and hence the metal remained in solution. The cooler, heavier Fluid A-B began to sink in the centre of the breccia complex through brine reflux, and established a 'donut-shaped' convection cell, with the hot Fluid A rising on the margins of the complex and the cold Fluid A-B sinking in the centre. The oxidised, acid, Cu-bearing Fluid A-B leached Cu from, and oxidised the stage 1 mineralised upper breccia complex as it descended. However, the interface with the ascending, less oxidised, hot, Cu-bearing Fluid A produced a redox and thermal front. At this front, Fluid A was cooled and Fluid A-B was reduced. They became Cu-saturated to release the metal in both, and precipitate it by overprinting stage 1 sulphides, as well as combining sulphate in the latter with Cu from both fluids to form new sulphides. This was stage 2 mineralisation which took place at 250 to 150°C. The spent Fluid A-B was then re-heated and pushed aside to join Fluid A, rising on the complex margin to close the convection cell. As this process proceeded, the 'hole in the donut' was progressively oxidised and leached to become the barren, hematite saturated HEMQ breccia. Fluid flow through the HEMQ breccia was optimum up to a composition of ~50% hematite, but then waned and was finally terminated when this had increased to 75% as its permeability was occluded, with only fracture controlled quartz and barite veins subsequently forming in the outer margins of that breccia. The result was a barren hematite breccia underlain and fringed by high grade Cu-rich and S-poor sulphides, zoned downward and outward from the redox front from chalcocite → bornite → chalcopyrite → pyrite, as explained in more detail below. During the stage 2 mineralisation, a proportion of fluids A and A-B escaped into the 'bedded clastic facies' and through faults to the surface to accommodate continuing inflow of Fluid A from below. Late in the system development, the inflow of the latter waned, the system cooled to Fe3+ hematite stable conditions and a hematite regime overprinted most of the magnetite altered column to depth. The occlusion of HEMQ and waning of inflow of Fluid A represents stage 3. The emplacement of the Olympic Dam deposit was entirely within an extensional regime, which in the lower to middle crust accommodated the emplacement of the voluminous deep magma chamber, and at surface produced the horst and graben setting of the OD red-bed Basin, into which the 'banded clastic facies' was deposited. This extension was responsible for the expansion of the OD Basin during development of the Olympic Dam Breccia Complex and mineralisation. It also led to downfaulting against a major fault-set resulting in the rotation of section of the 'banded clastic facies' and its underlying basement into the breccia complex. Alteration and mineralisation of these rocks had commenced prior to tilting and continued afterwards. As presaged above, following the cessation of stage 3 alteration, the deposit was periodically subjected to deformation, intrusive activity and fluid flow events that resulted in its partial erosion and preservation, and the addition and remobilisation of mineralisation, extending for more than a billion years into the Lower Cambrian. The latter, in particular, appears to have resulted in the redeposition, and deposition of significant additional, uranium below the Neoproterozoic unconformity.
The following is the detail and explanation behind the overview summary above:
The host to the Olympic Dam deposit, the Olympic Dam Breccia Complex, was developed within the undeformed, A-type, Roxby Downs Granite, which was emplaced at ~1593.87 ±0.21 Ma as part of the regionally extensive Hiltaba Suite magmatism (Cherry et al., 2018). The Roxby Downs Granite is interpreted to have been intruded into the overlying, broadly comagmatic, but marginally older, Gawler Range Volcanics. The latter were deposited at ~1594.73 ±0.30 Ma (Cherry et al., 2018) over the Palaeoproterozoic Wallaroo Group and ~1850 Ma Donington Suite granitoids. Both the A-type Roxby Downs Granite and bimodal, but predominantly felsic, Gawler Range Volcanics, which are anomalously rich in fluorine (McPhie et al., 2011), were emplaced in an intracontinental, extensional regime and represent a ~1595 to 1545 Ma large igneous province (Jagodzinski et al., 2021).
Mantle to crustal scale systematics - The hosts, alteration and mineralisation of the Olympic Dam deposit are part of a coherent and interrelated system of early Mesoproterozoic magmatism, hydrothermal alteration and deformation that extends for an up to 500 km width, from the western Gawler Craton to eastern Curnamona Province (Budd and Skirrow 2007; Skirrow et al., 2018; Reid 2019). According to Wade et al. (2019), such an extensive system requires the input of a vast amount of energy, which, on such a scale, can only be delivered into the crust from the mantle (e.g., Walter 2014). A study aimed at gaining an insight into the character of the mantle below the Gawler Craton is reported in detail in two papers by Wade et al. in 2019 (referenced below). This study was based on mafic rocks collected from the vicinity of significant IOCG and gold deposits/prospects, coming mainly from the mafic suite of the Gawler Range Volcanics, and filtered to exclude samples that have undergone crustal assimilation. These data indicate a mantle source for the mafic suite of the Hiltaba/Gawler Range large igneous province that is dominantly sub-crustal lithospheric mantle (SCLM) with varying magma compositions. These variations are interpreted to represent a vertically and laterally heterogeneous SCLM. In general, these rocks are characterised by enrichment in HFSE (high field strength elements) and LREE (light rare earth elements), negative Nb-Ta-Ti anomalies, Th/Nb ratios of <0.25 and variable Nd isotopic compositions. The geochemical data from samples taken from the Olympic IOCG Province form a distinct group, characterised by moderate to high Cr and Ni; general enrichment in HFSE including Zr, Nb, Th and Y; and moderate LREE enrichment. Sm-Nd isotopic compositions from the IOCG system samples are generally the most evolved. These characteristics are consistent with an SCLM source region that has been modified/metasomatised by subduction processes and was therefore hydrated, or partly hydrated mantle (e.g. Goodenough et al., 2002; Sandeman et al., 2003; Cai et al., 2013; Pearce et al., 2015; Condie and Shearer, 2017). Subduction and metasomatism have been recognised as being important processes that generate metal enrichment in the mantle (Richards 2009; Groves et al., 2010; Tassara et al., 2017; Xu et al., 2017), resulting in residual siderophile and chalcophile-rich zones within the lower SCLM, a potential viable source of Cu and Au.
Wade et al. (2019) indicate the isotopic composition of these magmas from the eastern Gawler Craton suggest subduction may have been as early as the late Neoarchaean, or crust of that age reworked during the Palaeoproterozoic. However, the current craton margin parallels the inferred active margin of the ~1850 Ma Cornian Orogeny and the suture between the two cratonic masses involved that were amalgamated into a single block during the 1730 to 1690 Ma Kimban Orogeny. The remnants of these two cratonic masses now constitute the Gawler Craton and Curnamona Province respectively, separated by the Neoproterozoic Adelaide Rift Complex which reactivated the Palaeoproterozoic suture during the Neoproterozoic. These two earlier collisional subduction events may well have left a legacy fertilised sub-crustal lithospheric mantle below the Olympic IOCG Province.
With minor variations on that proposed by Wade et al. (2019), a model is suggested, involving:
i). a rising mantle plume generated the extensional regime that accompanied the Hiltaba/Gawler Range large igneous province event; or alternatively, regional extension led to delamination and detachment of the lower asthenospheric mantle and the decompression melting and upwelling of asthenospheric mantle;
ii). the hot plume/upwelling asthenosphere is envisaged to have preferentially partially melted the more susceptible hydrated, metasomatised lithospheric mantle (SCLM), but also to a lesser degree the less- or un-metasomatised, anhydrous adjacent SCLM;
iii). this partial melting produced hydrous and oxidised mafic/ultramafic magmas, pressure mixed with voluminous 'hydrous fluids' as a magmatic volatile phase, containing H2O, Cu, Au, Fe and F stored in SCLM that had been metasomatised during the earlier Cornian and Kimban orogenies;
iv). oxidised felsic magmas of the Hiltaba Suite were produced by partial melting of lower crust by the mafic-ultramafic magmas and plume, and in part by mixing with fractional crystallised phases of the mafic magmas;
v). the mafic to ultramafic magmas ascended into the crust to their level of neutral buoyancy, where they differentiated and cooled, accompanied by boiling that released the pressure-mixed magmatic volatile phase which collected in the roof of the magma chamber, at or near the ductile-brittle transition.
vi). the magmatic volatile phase-fluid, composed of hydrous, oxidised, Fe- and F-rich fluids containing elevated Cu-Au, was tapped where the roof of the magma chamber was breached by major transcrustal brittle structures (e.g., the Jubilee Fault) and ascended to contribute to the formation of IOCG mineralisation at shallow levels.
NOTE: The higher SG mafic to ultramafic melts were mostly trapped at their level of buoyant equilibrium in the lower crust, while lighter crustal contaminated and volatile rich mafic magma (e.g., lamprophyres), and the lower SG felsic magmas continued to shallower levels. In contrast, the overpressured fluids followed available, mainly structural dilational pathways towards the surface followed by light, fluid- and gas-saturated mafic to ultramafic magmas.
Mantle to deposit scale connection - The vertical and lateral heterogeneity of the SCLM below the Olympic IOCG Province is also supported in geophysical data by the presence of domains with high conductivity (seen in MT data), and coincident 'bland seismic character' within more extensive background zones of low conductivity and well developed seismic texture. These geophysical anomalies illustrate a convincing connection between the lower lithospheric mantle and the Olympic Dam deposit, and are described in the main Geophysical Expression section above. They are also consistent with the model proposed in the previous paragraph (after Wade et al., 2019). Regionally extensive, deep, conductive and bland seismic domains, which persist over lengths of hundreds of kilometres, are interpreted to reflect zones of Palaeoproterozoic (or possibly late Neoarchaean) subduction imposed metasomatism within the lower lithospheric mantle, that have been reworked and partially melted during the early Mesoproterozoic by a major heat source, as detailed above. The re-melting of the earlier subduction imposed metasomatic zones is interpreted to have produced extensive, hydrated, Cu-Au-F fertile altered zones whose conductivity is influenced by water (as OH-), fluorine and iron minerals. Upward, within the upper lithospheric mantle and lower crust, similar conductive and bland seismic domains are interpreted to reflect alteration generated by the passage of these fertile mafic to ultramafic magmas and large volumes of un-mixed magmatic volatile phase fluids. Such magmas and fluids are envisaged to have inherited metals and volatiles stored in the fertile, pre-existing subduction metasomatised lithospheric mantle. It is also likely that the highly corrosive, fluorine-rich, metalliferous magmatic volatile phase fluids thus generated, scavenge further metals, particularly Fe oxides, from the lithospheric mantle and crust during their ascent, as well as creating this alteration. The upper conductive and bland seismic domain is consistent with ponding of the mafic to ultramafic magmas and magmatic volatile phase fluids, up to the ductile-brittle transition in the crust at ~15 km, as described above. The pregnant fluids were then focussed into brittle, structurally controlled conduits, emanating from that ponded reservoir. These conduits are interpreted to be reflected by more restricted, but well-defined, 5 to 10 km wide 'fingers' of the same conductive-bland seismic character emanating from the considerably more extensive underlying domain. These fingers are clearly shown to pass up into the Olympic Dam and other IOCG deposits and significant prospects within the Olympic IOCG Province as described above in the Geophysical Expression section. The 'finger' that underlies Olympic Dam is one of the most intensely conductive and has a width at the Neoproterozoic unconformity of the same order as the Olympic Dam Breccia Complex into which it passes.
The ~50 km2 and 500 to >1000 m thick Olympic Dam Breccia Complex embraces and hosts the Olympic Dam deposit. The texture, contact relationships, distribution and non-stratified character of the breccias of the complex have been interpreted to be consistent with subsurface fragmentation of already solid granite, caused by a combination of tectonic and hydrothermal processes (Oreskes and Einaudi, 1990; Reeve et al., 1990; McPhie et al., 2011; Ehrig et al., 2021). The deposit and the complex appear to be centred on the intersection of the ENE to NE-SW trending regional Jubilee (or Masher's) Fault and the postulated pre-mineral NNW-SSE fault that is inferred to also control the elongation of the mineralised zone. The core of the complex is dominated by breccias containing clasts of the Roxby Downs Granite the Gawler Range Volcanics, and the younger 'bedded clastic facies'. The latter had been deposited at ~1590.97 ±0.58 Ma (Cherry et al., 2018) in a fault bounded sedimentary basin, overlying both the Roxby Downs Granite and Gawler Range Volcanics at Olympic Dam (McPhie et al., 2016). This basin is most likely one of a number of interconnected early fault controlled depocentres in the evolution of the ~1575 to 1400 Ma Cariewerloo rift basin into which the Pandurra Formation (dated at one location as ~1424 Ma Beyer et al., 2018) was deposited. A wedge of sandstone interpreted to belong to the Pandurra Formation is structurally interleaved with the 'bedded clastic facies' within the Roxby Downs Breccia Complex. The provenance of granitoid detritus in the 'bedded clastic facies' is interpreted to most likely be the Roxby Downs Granite. This would require cooling and partial exhumation of the granite prior to ~1591 Ma (Cherry et al., 2018), most likely during the ∼3 m.y. gap between granite emplacement and deposition of the 'bedded clastic facies'. The southeastern lobe of the deposit is also characterised by the presence of substantial wedge shaped blocks of 'bedded clastic facies' rocks that have been tilted to near vertical dips and down-faulted into the Olympic Dam Breccia Complex in an extensional stress field. These blocks have vertical and horizontal dimensions of the order of 1 to 2 km and hundreds of metres in thickness. They have been brecciated, altered and mineralised and are overprinted by the most intense HEMQ hematite-quartz breccia alteration phase, at least in part following down-faulting. However, McPhie et al. (2016) also consider it likely that the 'bedded clastic facies' were deposited while the Olympic Dam hydrothermal system was active. This implies long-lived hydrothermal activity most likely involving repeated episodes.
There is evidence within the Olympic Dam Breccia Complex that the interpreted deep mantle derived fluids i). are oxidised and rich in Fe, as indicated by the oxidation and intense iron oxide alteration and replacement of the hosts; and ii). contain anomalous fluorine and are very corrosive with a low pH. The latter is consistent with the high relative fluorine content of altered rocks and strong chlorite and sericite to advanced argillic alteration of the breccia complex. It is also emphasised by the vast amounts of silica produced by the alteration of silicate minerals that has been removed during alteration, as well as the corrosion and dissolution of free silica/quartz from the host rocks (as outlined in the Alteration section above).
The development of the Olympic Dam Breccia Complex is envisaged as follows, largely after McPhie et al. (2011) and Cherry et al. (2017):
• Deposition of the bimodal Gawler Range Volcanics at ~1594.73 ±0.30 Ma over the Palaeoproterozoic Wallaroo Group and ~1850 Ma Donington Suite granitoids, was followed by intrusion of the Roxby Downs Granite at ~1593.87 ±0.21 Ma, all within an extensional regime;
• Soon after deposition and cooling to a brittle state, a regional deep seated fault system propagated into the Roxby Downs Granite, and initiated structurally controlled brecciated at the intersection of major, steep, ENE to NE-SW and NNW-SSE fault sets. These were trans-crustal structures, which tapped the reservoir of cogenetic un-mixed deep crustal fluids and volatile-rich mafic to ultramafic melts ponded near the ductile-brittle transition at a depth of ~15 km. Both the melt and the hydrated, oxidised, iron, fluorine, Cu and Au rich fluids were channelled upwards via the dilational, structurally brecciated fault intersection. These overpressured fluids, ascended through the fractures of the mechanical breccia, replacing wallrocks, creating an upward migrating replacive breccia, the nascent Olympic Dam Breccia Complex. It is suggested, that while some of the fluid pressure may have been periodically relieved to surface by movement on the major faults, the fluid system was still predominantly under overpressure. This overpressure facilitated hydraulic brecciation and inflation (natural fracking) along weaknesses imposed by the fault system stress field, allowing the maximum access of fluids to develop the replacive breccia.
• Displacement on these fault systems in an extensional stress field also initiated changes in the topography at surface to produce local uplift and erosion from horsts and deposition in an associated graben (the 'OD Basin'), in a 'basin and range setting. As a consequence, the Gawler Range Volcanics and then the Roxby Downs Granite were exposed and by ~1590.97 ±0.58 Ma supplied detritus into the OD Basin to deposit the red and green mudstones and sandstones of the 'bedded clastic facies', whilst distal eruptions contributed tuffaceous mudstone. During this period, the breccia complex had propagated into the base of the Gawler Range Volcanics, as had the volatile and gas-rich ultramafic to mafic dykes that had accompanied the deep hydrothermal fluids in their ascent. At this stage the hydrothermal brecciation dominated over the structural fracturing.
• Continued extension initiated the Woodall and Woodall Jr. faults, and deepening of the OD Basin graben. The pronounced downthrow on the northern side of these faults led to the flat lying lower sections of the semi-consolidated 'bedded clastic facies' strata being tilted and collapsing 'from either side', first as a down-warp, then as fault controlled wedges, into the accommodation space generated by the extension. Meanwhile, at the basin floor level, debris flow conglomerates were deposited from the steeper fault controlled scarps of the graben as part of the ongoing deposition. During this stage, the breccia complex encroached into the lower 'bedded clastic facies' and the hot mantle derived hydrothermal fluids mixed with cold basinal brines. It is likely that this breakthrough from the confining granite and volcanic rocks, into the relatively shallow, permeable, cooler and more oxidised red bed sedimentary basin may have resulted in a change from the earlier higher temperature, pressure and less oxidised conditions.
• As the overall regime described above evolved, fluid flow from below continued to produce progressively more intense alteration and 'replacive brecciation' affecting the Roxby Downs Granite, Gawler Range Volcanics and 'bedded clastic facies', including brecciation, dissolution of contained volatiles and internal alteration and mineralisation of the mantle sourced mafic to ultramafic dykes that accompanied that fluid upward from the apex of the parental magma chamber at depth.
• As the overlying OD Basin matured in a continuing extensional setting, it was amalgamated with other localised interconnected grabens, and become part of the developing, broader, ~1575 to 1400 Ma Cariewerloo rift basin, in which, possibly after a hiatus, the more widespread Pandurra Formation was deposited. Late tectonic activity along the Woodall faults resulting in slivers of that sandstone also collapsing into the breccia complex with underlying units of the upper 'bedded clastic facies'. The down-faulting of the deposit and deposition of the 400 to 2000 m thick Pandurra Formation in the ~420 x 170 km Cariewerloo red bed basin, concealed and preserved the deposit from erosion. Dating of uraninite within the deposit has yielded a small number of dates at ~1.44 Ga, which are taken to reflect minor remobilisation related to the Late Kararan Orogeny that involved reactivation of major regional shear zones and commenced the inversion of the Cariewerloo Basin (Ehrig and Clark, 2020).
The evolution of the breccia complex as outlined above, the inter-relationship of lithologies, structural framework, and the character of the rocks, all suggest the development of the Olympic Dam Breccia Complex involved a relatively long lived, progressive, pulsed, but reasonably passive process, at shallow depths, most likely punctuated by localised small scale phreatic bursts. This is implied by:
i). Depth - although the hydrothermal system has roots suggested to extend to the brittle-ductile transition at ~15 km depth, and from there to the mantle, the formation of the deposit is indicated to have principally taken place at between 500 and 2000 m below the contempory surface. The lower limit, taken here is the lowest >5 wt.% Fe granite to hematite breccia lithofacies which occurs at a depth of <1.5 km below the base of the >500 m thick 'bedded clastic facies', the top of which was the sedimentary basin floor. Interpreted exhalative chemical precipitates within the 'bedded clastic facies' and sub-surface boiling textures in the deposit are additional evidence the system evolved at a depth of <1 to 2 km and interacted with the overlying active OD Basin (Corriveau et al., 2022). Subsequent structural attenuation may have thinned this interval which is underlain and fringed by a greater thickness of less intense alteration on the margins of the system.
ii). Temporal extent - the development of the deposit is inferred to have continued over much of the interval in which the 'bedded clastic facies' were deposited, and continued as they collapsed into the breccia complex, as outlined previously.
iii). Passive setting - during this period, apart from along scarps on the basin margin where conglomerate-breccia wedges formed, deposition of the directly overlying 'bedded clastic facies' produced a thick sequence of laminated to finely bedded mudstone and sandstone, albeit gently to moderately slumped, even while the breccia complex was encroaching into the lower members of that facies <500 m below. A dynamic, venting breccia zone would be expected to produce a chaotic mélange.
iv). Replacive brecciation - in the early stage of brecciation, clasts of the breccia are observed to comprise horses of the protoliths bounded by fault induced fracture margins which were then progressively replaced inwards from those fractured margins until only their ghosts remain (Ehrig and Clark, 2019)
v). Collapse - clasts within the breccias appear to largely be of in situ origin, composed of lithologies either from the same level at which the breccia is formed, or are 'exotic', predominantly from stratigraphically higher units. This implies continued replacive brecciation, followed by structural and solution collapse brecciation. The latter could be explained by volume reduction through alteration and removal in solution of components such as silica by the corrosive hydrothermal fluids and hydrolytic alteration. This volume reduction, coupled with accommodation within the extensional regime which also resulted in physical collapse of large wedges of 'bedded clastic facies' from higher in the system. The extreme alteration in the core of the system produced disaggregation of the protolith and degradation of clasts to progressively finer particle sizes. This is envisaged as culminating in fluidisation of the matrix, allowing mixing of clasts during a long lived continuation of repeated fluid flow pulses. However, clasts of early magnetite alteration are seen, overprinted by chalcopyrite ±pyrite mineralisation, and preserved within a low temperature K-Fe altered granite breccia (e.g., Corriveau et al., 2022). These large clasts are interpreted to have been transported vertically upwards and incorporated into parts of the shallower alteration facies, although they may represent relicts of early in situ prograde magnetite alteration, overprinted by later retrograde hematite-sulphide facies.
Deposition of Mineralisation
The transport and deposition of copper in almost all deposit types is governed by both the metal's availability and solubility. There are four main variables that singly or in combination determine the solubility of copper, resulting in saturation and partial to total release from solution: i). temperature and pressure, where the metal remains in solution until one or both are sufficiently reduced, e.g., in porphyry Cu deposits; ii). pH, whereby Cu is held in solution at low pH until the transporting fluid is neutralised/diluted, e.g., in supergene blankets; iii). Eh - where Cu remains in solution in an oxidised regime, until more reduced conditions are encountered, e.g., sediment hosted Cu deposits; iv). interaction with reactive rocks/minerals/fluids/gases that extract Cu from solution; these include in order of declining reactivity: sulphates/sulphides/and 'other sulphur anions'; carbonates; mafic minerals; feldspars. It can be argued that all of these four controls were active in parts of the Olympic Dam mineralised system.
Among all of the detail described in this summary, a number of key observations seem important when considering the potential processes involved in the formation of the deposit, as detailed, with source references, above. They are:
• The sulphur content as sulphides, which decreases upwards from deep in the Roxby Downs Breccia Complex to the base of the hematite-quartz-barite HEMQ breccias, or the Neoproterozoic unconformity peripheral to the flanks of the latter. This corresponds to an upward transition from pyrite (~53% S) → chalcopyrite (~35.0% S) → bornite (~24.5% S) → 'chalcocite' (~20% S) from the base to top respectively. However, as Cu grades in the chalcocite zones are 2 to 3 times those that are predominantly chalcopyrite, the total sulphur content is not significantly lower. Never-the-less, overall, the sulphur content of the system increases downward and persist with depth, implying it was largely derived from below.
• The copper content is generally highest adjacent to and within ~200 m below the lower and lateral contact of the HEMQ breccias, and gradually decreases away from that contact, corresponding to the mineralogical transition from chalcocite → bornite → chalcopyrite → chalcopyrite-pyrite → pyrite, i.e., from minerals that contain the most to the least copper per atom of sulphur. This implies that, at least in part, Cu was supplied from above.
This conclusion is based on experience in other mineralised systems, particularly hypogene sediment hosted deposits and supergene enrichment (e.g., of porphyry Cu deposits). These systems display a transition across a redox boundary, from an oxidised setting to reduced conditions with a zonation (in part or in full) from chalcocite → bornite → chalcopyrite → pyrite. This zonation can, in those systems, be shown to represent the passage of Cu-bearing fluids from the oxide setting towards the outer pyrite zone, with the highest grades and Cu:S ratios adjacent to the redox boundary and the lowest distal to that interface. This is related to the properties of Cu and should equally apply to IOCG systems. It reflects the progressive addition of Cu to the sulphide lattice, which is most pronounced from closest to the source, and least, distal to that same source. This process is usually accompanied by the expulsion and sometimes associated deposition of iron oxides.
• Two ore types have been recognised within the Olympic Dam Breccia Complex. These are:
i). High grade, >2% Cu equivalent, lower tonnage (~1 Gt) ore, occurring as a series of structurally focussed, discrete ore shoots that have a strongly anisotropic and variable geometry, as well as a restricted, but similarly anisotropic halo of ~1% Cu disseminated sulphides. This mineralisation is hosted in fluorite-rich hematite breccias containing bornite-chalcocite, with lesser chalcopyrite. It is accompanied by intense brecciation and a high rate of fluid-rock interaction where chemical dissolution is > physical brecciation; and
ii). Low grade, >0.6% Cu equivalent high tonnage (~10 Gt), disseminated chalcopyrite (-pyrite) ore. This mineralisation is reasonably homogenous and spread throughout the complex, but principally below the high grade ores, with a slight increase in grade towards the centre of the deposit.
• The hematite saturated, sulphide free, 'barren', hematite-quartz-barite (HEMQ) breccias are the most intensely altered and oxidised rocks in the Olympic Dam Breccia Complex, albiet formed at the lowest temperatures. They represent a regime in which copper is the most soluble and hence, if present may not be precipitated. These breccias, however, are generally closely flanked laterally and downward by the highest grade, highest Cu:S ratio minerals (chalcocite-bornite), which decrease in both grade and Cu:S ratio away from a broad zone adjacent to that contact (Reynolds, 2000). Uranium mimics the distribution of copper, whilst significant LREE mineralisation, and some of the higher grade gold is concentrated between the uraniferous bornite-chalcocite mineralisation and the ‘barren’ HEMQ core of the deposit, straddling either side of the contact with the latter.
• Two different geochemical associations, or 'IOCG signatures', have been recognised within the IOCG mineralisation of the Olympic Dam Breccia Complex, with separate, distinct, spatial distributions: i). Cu-U3O8-Se-S, the main economic and closely associated elements, have a concentric zonation, outward and downward from the base of the HEMQ breccia. ii). Au-W-Mo-Sb-As, which forms a vertical, >1800 m deep elongate column, still open at depth, in the southeastern lobe of the deposit, spatially coinciding with hematite (>5% Fe) and volcanic breccias within the Olympic Dam Breccia Complex. It is especially evident at depths below 1200 m, as well as in generally shallow quartz-barite-hematite breccias (HEMQ) and hematite-quartz 'bedded clastic facies' sediments (KHEMQ). This vertical mineralised column is considered likely to be related to the major subvertical WSW-ENE striking fault system acting as a hydrothermal-fluid conduit. However, the concentration and zoning of the first group, while being controlled by the same mineralisation/alteration corridor, appears to overprint and be spatially independent of the Au-W-Mo-As-Sb association, i.e., two separate mineral associations taking advantage of the same conduit.
Although the available interpretations and literature suggest the HEMQ represents the outflow of a spent mineralising hydrothermal fluid following ore deposition (e.g., Ehrig and Clark, 2020), another variation might be considered as follows.
It is suggested the formation of the Olympic Dam IOCG-U-Ag deposit involved the interaction of three fluids, based on observations recorded at Olympic Dam, as described and cited above, and from similar deposits/prospects within the same district:
Fluid A - is the ascending hydrothermal fluid suggested to have been derived from parental fluid generated during partial melting of pre-existing hydrous, subduction metasomatised and fertilised, mantle lithosphere. This parental fluid then ascended, pressure-mixed with mafic to ultramafic magmas, to be ponded near the ductile-brittle transition in the crust, as detailed in the Geophysical Expression section above. The apex of that very large ponded reservoir at ~15 km depth is seen as having been tapped via deep brittle structures that have channelled a segregated fraction of that fluid upwards, accompanied by volatile-laden mafic to ultramafic magmas. As such it is derived from a magmatic mass of hundreds of km3, rather than having unmixed/degassed from magmatic bodies of maybe several km3 within of shallowly below the ore deposit. Such a large system is essential to providing the tonnage of Cu at Olympic Dam. However, because of their common origin, the volatile-rich ~1590 Ma mafic to ultramafic magmas will also carry similar elements and fluids which will unmix/degas at shallower depths and also contribute to the deposit. Fluid A is seen to have been very hot (having deposited a biotite-magnetite-K feldspar assemblage deep in the Olympic Dam Breccia Complex at >400 to 600°C). It was also overpressured, as suggested by the hydraulic opening of a denser array of fractures related to the prevailing stress field, with contemporaneous, inward progressive alteration of the blocks of granite between those fractures. Based on the associated alteration, the fluid had an intermediate to reduced oxidation state, carrying appreciable Fe2+, (e.g. as aqueous FeCl2; Crerar and Barnes, 1976; Seyfried and Ding, 1993) which will tend to limit its sulphur carrying content (H2S >> ΣSO42-) to relatively low levels (Skirrow, 2022). It is hydrated, rich in volatiles, particularly fluorine, and carried anomalous quantities of Cu, U, Au and Ag, all derived from re-melting of previously subduction fertilised lithospheric mantle. High temperature fluids of this type found within the Olympic Dam district carry 2 to 4 wt.% Cu in some fluid inclusions, which including verified chalcopyrite. If chalcopyrite-bearing inclusions are excluded, these fluids still carry 300 to 600 ppm Cu (Bastrakov, Skirrow and Davidson, 2007).
The sourcing of ore forming fluid from a very large, deep, differentiated parental magma chamber, is directly analogous to what is now understood to produce porphyry Cu deposits, but with differently sourced felsic magmas. In porphyry systems, the segregated fluids, sourced from the apex of the deep parental magma chamber, are accompanied by fluid saturated porphyries derived from the same part of the batholith which contribute mineralisation in the deposit via the pre-solidus A-veins, while the bulk of the ore is provided by the deeply sourced fluid, occurring as B, C, D, etc., veins cutting the solidified porphyry and country rock. As such, the mineralised ~1590 Ma mafic/ultramafic rocks at Olympic Dam would be analogous to the intrusive stocks of a porphyry system.
The main point of difference between porphyry and IOCG deposits, is that in the former, other than the early A-veins, the bulk of mineralisation occurs as discrete veins, whilst even the disseminated sulphides are in now closed microfractures. This is the product of overpressured fluids facturing a brittle host. In contrast, IOCG deposits often maintain a porosity within the matrix that accommodates the passage and deposition of fluids, with veins at Olympic Dam largely confined to the fractured granites and volcanic rocks external to or as horses within the breccia complex, and late following the occlusion of porosity and solidification (e.g., the carbonate veins).
Fluid B - is a cool, oxidised basinal brine, accumulated in an arid to semi-arid intra-continental red-bed basin; the OD Basin into which the 'bedded clastic facies' were deposited. This basin is interpreted to be fault controlled, related to the same structural network that controlled the ingress of Fluid A. The 'bedded clastic facies' are overlain by the Pandurra Formation deposited in the succeeding Cariewerloo rift basin. It is likely that the 'bedded clastic facies' were deposited in one of an interconnected network of 'basin and range' type grabens and horsts of the type that usually precede the development of rift basins. This is supported by the recognition of other similar sedimentary sequences of the same age distributed along the eastern margin of the current Gawler Craton (Ehrig and Clark, 2020). Bastrakov, Skirrow and Davidson (2007) describe fluid inclusions that suggested oxidised, lower temperature, SO4-rich brines formed in these basins and were involved in the development of the hematite alteration in the deposits of the Olympic Dam IOCG Province. This is also supported by the observation by Oreskes and Einaudi (1992) that the hematite associated ore-bearing breccias were deposited by fluids of significantly lower temperature and lower δ18O values than Fluid A.
Fluid A-B - a hybrid fluid resulting from the mixing of fluids A and A-B, initially in the apex of the Olympic Dam Breccia Complex, when it finally breached the base of the OD Basin, and then in the permeable sands of the 'bedded clastic facies'. The composition and temperature of this hybrid fluid will vary considerably, depending on its location relative to the two end members.
Based on observations and interpretations in a range of sources, including Skirrow (2022), Reid (2019), Dmitrijeva et al. (2019), Schlegel et al. (2017), Ehrig et al. (2017), Krneta et al. (2017), Bastrakov, Skirrow and Davidson (2007), Haynes et al. (1995), Oreskes and Einaudi (1992), and other references listed previously, the Olympic Dam deposit may have initially formed as follows:
• Fluid A was tapped from the apex of the deep mafic to ultramafic magma reservoir and channelled up the regional, transcrustal Jubilee Fault, focussed along its intersection with a NNW-SSE fault that was to control the elongation of the Olympic Dam deposit. This occurred following solidification of the Roxby Downs Granite, after ~1593 Ma.
• Brecciation and alteration - the fluid column within the fault controlled channel hydraulically transmitted the deep llithostatic pressure to shallower levels to open and inflate fractures initiated by the prevailing stress field (natural 'fracking'). This allowed ingress of the highly corrosive hydrofluoric acid rich Fluid A into those fractures to decompose and alter the inter-fracture Roxby Downs Granite. This initiated the development of the Olympic Dam Breccia Complex as both a mechanical and replacement breccia. In so doing, it appears to have produced an early albite-actinolite ±magnetite alteration, overprinted by a biotite-magnetite-K feldspar assemblage at temperaures of >400 to 600°C. During the same period, the 'bedded clastic facies' were being deposited within a basin controlled by the fault regime related to the ingress of fluids. As such those faults may have periodically relieved the overpressure and allowed escape of Fluid A to shallower levels, to percolate within, sub-surface, and into at surface, the sediments of the OD Basin, particularly the hematitic mudstone and sandstone of the KHEMQ unit, implanting an IOCG signature.
• Upward migration and stage 1 mineralisation - the Olympic Dam Breccia Complex is interpreted to have generally passively expanded upward and outward through the Roxby Downs Granite and into the overlying Gawler Range Volcanics. During this period, alteration continued to evolve, producing potassic alteration, progressing to assemblages of magnetite-fluorapatite-ankerite-calcite and siderite-chlorite-quartz. This alteration was accompanied by weak Cu sulphides and uranium, possibly to grades of 0.1 to 0.3% Cu, typical of the magnetite rich early stage mineralisation found elsewhere in the Olympic IOCG Province. The precipitation of sulphides during this stage was initiated by progressive cooling, and took place in the magnetite stability field of ~450 to 350°C as the fluid ascended, likely enhanced by periodic release of overpressure via the major faults. However, at these temperatures, Cu was only mildly exceeded saturation, insufficient to promote significant exsolution, and in conjunction with the low levels of available sulphur in the fluid, left substantial excess Cu in the residual Fluid A. This stage of mineralisation was restricted to a pyrite → pyrite-chalcopyrite assemblage and is interpreted to correspond to the Au-W-Mo-Sb-As 'IOCG signature' seen from the lowest to highest development of alteration within the Olympic Dam Breccia Complex. This signature is also seen in the red sandstone facies of the 'bedded clastic facies' where fluids may have escaped to surface or near surface in the faults bounding the OD Basin, as discussed above.
• Breakthrough into the OD basin and transition to hematite stability - the Olympic Dam Breccia Complex is interpreted to have breached the capping Gawler Range Volcanics into the 'bedded clastic facies'. This led to the mixing of fluids A and B, a reduction in pressure regime, lowering of temperature and possibly an increase in the upflow of Fluid A, as well as the addition of solutes. This mixing had two main consequences: i). a sharp decrease in temperature caused the acidic, Cu-bearing Fluid A to transit from high temperature Fe2+ magnetite-stable conditions to Fe3+ hematite stability, enhanced by mixing with the cold and strongly oxidised Fluid B; ii). the addition of solutes from Fluid B, particularly SO42- and brines generated in the red bed basin. The mixing of these two fluids formed the hybrid Fluid A-B. While the reduction in temperature decreased the solubility of Cu, this was compensated by the increase in oxidation state, maintaining the metal in solution.
• Convection - the hybrid Fluid A-B was a much cooler brine, and as such, was denser than Fluid A. As a consequence, it began to reflux, sinking in the centre of the breccia complex where it displaced ('pushed aside') the hotter ascending Fluid A, to form a NNW-SSE elongated, 'donut shaped' convection cell. The sinking Fluid A-B caused the rising hot Fluid A below to be displaced laterally on the underside of the donut, towards the outer margins of the Olympic Dam Breccia Complex, thus widening the shallow complex on its margins. It then ascended around the outer and upper side of the donut to encounter Fluid B above, formed the cool Fluid A-B, and then migrate toward the centre of the system, where it refluxed, down through the 'hole in the donut'. The cooler, sinking, Fluid A-B in the centre of the 'donut' was eventually heated at depth by the ascending Fluid-A, and began to rise again on the margins of the complex with the latter.
• Stage 2 mineralisation - the Cu-rich and SO42--bearing Fluid A-B was strongly oxidised and incapable of releasing the residual Cu inherited from Fluid A. As it sank through the 'hole in the convection donut', it was sufficiently acidic to supplement its load by stripping additional metal from breccias of the upper stage 1 mineralised Olympic Dam Breccia Complex, whilst oxidising the leached host rock, converting magnetite to hematite. This process initiated the formation of the HEMQ hematite-quartz-barite breccia which became increasingly hematite-rich, and grew to fill the 'hole in the donut'. However, at the interface with the upwelling, hot, less oxidised, ferrous iron (Fe2+) stable, magmatic-hydrothermal Fluid A, the strongly Fe3+ oxidised Fluid A-B was reduced. Under these conditions it could not retain its contained metal load in solution, which was then released. Multiple processes, or combinations thereof, took place at this interface, which represented a downward and outward advancing redox front. Because this redox front was between two fluids, its location varied due to temporal difference in the relative flow rate of the two fluids and the porosity of the country rocks at any one point. As a consequence it was irregular. The processes and reactions that deposited Cu sulphides at this front included:
i). Released Cu from Fluid A-B reacted with pre-existing stage 1 pyrite and chalcopyrite to progressively produce chalcopyrite, bornite and eventually chalcocite. However, stage 1 sulphides continued to be deposited by the ascending Fluid A, below and lateral to the stage 2 mineralisation, beyond the immediate influence of the descending Fluid A-B.
ii). Exsolved Cu and SO42-, both from Fluid A-B, combined to precipitate Cu sulphides;
iii). Remaining Cu in the ascending Fluid A, upon being cooled by Fluid A-B, reacted with the SO42- of the latter, as well as stage 1 mineralisation to form further sulphides;
iv). Reactive mafic to ultramafic (lamprophyre) dykes, which are estimated to occupy ~30% of the Olympic Dam Breccia Complex, caused Cu to be released from both fluids A and A-B, in the presence of a sulphur source, to produce Cu sulphides, and from Fluid A alone, deeper in the mineralising system. These magmas, which were likely also saturated with metal and volatiles will have a). contributed to the heat budget; and b). released and precipitated additional Cu sulphides internally and, when reacting with fluids A and A-B, on their margins.
Gold is also carried in fluids A and A-B, and has a solubility that is similarly strongly influenced by Eh, but to a lesser degree than Cu. As a consequence, it is deposited inboard of Cu, straddling the oxidation front.
These stage 2 reactions took place at temperatures of 250 to 150°C (Skirrow, 2022, Skirrow, 2010, Bastrakov, Skirrow and Davidson 2007). They were most prevalent within a transition zone of 100 to 500 m outboard of the advancing redox front, with the Cu grade rapidly increasing ahead of that front, to a peak characterised by a chalcocite-bornite assemblage. Past this zone of maximum Cu deposition, both the amount of available, unreacted Cu and SO42- contributed by Fluid A-B declined. As a result both the Cu grade and Cu:S ratio also progressively declined, with the transition from chalcocite → bornite → chalcopyrite-pyrite → pyrite. From the Cu grades, Cu:S ratios of mineralisation, and the general upward decline in S content, it would appear Fluid A-B only made a relatively minor sulphur contribution, with the bulk being from the stage 1 sulphides that were partly overprinted and rimmed by later phases, and partially dissolved and redeposited. However, Bastrakov, Skirrow and Davidson (2007) note that sulphur in both stage 1 and stage 2 alteration assemblages from deposits and sub-economic prospects in the Olympic Dam district was derived either from cooling magmas and/or crystalline igneous rocks carried by relatively oxidised fluids (ΣSO42- ≈ ΣH2S, δ34Ssulphides from –5 to +2‰) or from crustal sedimentary rocks (δ34Ssulphides from +5 to +10‰).
Ehrig and Clark (2020) note that the high grade (stage 2) mineralisation is often aligned parallel to the late flat (listric), extensional fault set in the upper parts of the deposit (Fig. 3). This may reflect earlier, now annealed, syn-mineral, fault set related to the extensional regime operating during mineralisation.
Stage 2 mineralisation was accompanied by lower temperature retrograde hydrolytic hematite-sericite-chlorite-carbonate alteration, and strong silica depletion. Fluid A-B continued to descend, altering the country rock, before being diluted and heated by Fluid A, then rising on the margins of the complex as part of the 'donut shaped' convection cell. During stage 2, ingress of Fluid A continued to contribute metal and other solutes to the system. This had to be balanced by upward or outward fluid loss responsible for the shallow sub-surface boiling textures and epithermal veins in sections of the system. The stage 2 mineralisation was responsible for the Cu-U-Au-Ag 'IOCG signature' that accompanies and overprints the Stage 1 Au-W-Mo-Sb-As signature.
The redox front represented by the lower and lateral margins of the leached, sulphide-free HEMQ breccia was not static, but advanced outward and downward with the continuing flow of Fluid A-B, scavenging high grade stage 2 chalcocite-bornite mineralisation in its path, leaving barren HEMQ in its wake, and reinforced mineralisation ahead; basically sweeping a progressively higher grade wave of mineralisation ahead of its advance. Silica leached during the hydrolytic alteration and transported by the spent Fluid A-B within the convection cell was deposited in veins on the margin of the Olympic Dam Breccia Complex and within the HEMQ breccia forming a silica-hematite cap, whilst SO42- contributed by Fluid B was involved with the deposition of sulphates, particularly barite in the HEMQ breccia core.
High grade stage 2 mineralisation gradually decreased in intensity as the deposition of hematite progressively increased within the HEMQ breccia occluding its porosity. Fluid flow through the HEMQ breccia was optimum up to a composition of ~50% hematite, but then waned and was finally terminated when this had increased to 75%, with only fracture controlled quartz and barite veins subsequently in the outer margins of that breccia.
• Stage 3 alteration/mineralisation - which took place in the waning stages of the mineralised system. The temperature of Fluid A was progressively reduced, and the hematite to magnetite stability front descended, whilst the stage 2 convection cell closed down. This resulted in hematite further overprinting earlier alteration assemblages and the cessation of significant mineralisation.
'Bedded Clastic Facies'
Prior to deposition of the stage 2 mineralisation, the Olympic Dam Breccia Complex had broken through the roof of the Roxby Downs Granite and the Gawler Range Volcanics to encroach up into the 'bedded clastic facies'. As detailed above, fluid connectivity was then established between the breccia complex and the permeable units of the 'bedded clastic facies', allowing mixing between fluids A and B and limited deposition of mineralisation within the sedimentary rocks of the OD Basin. The rocks of the 'bedded clastic facies' were mostly above or lateral to the main mineralising domain, and as such have not been brecciated and altered to the degree of the bulk of the rocks of the Olympic Dam Breccia Complex. Prior to, and following this event, it is likely that tectonic activity allowed periodic escape of Fluid A from the breccia complex up major structures, such as the Masher's and Woodall faults, resulting in ingress into permeable units in the OD Basin above the breccia complex, and hot springs on the basin margin feeding fluid and solutes into the basin. In both cases this would have resulted in hematitic alteration, particularly of the KHEMQ hematite-quartz-rich sandstone and red mudstone, and imparting the IOCG signature on that unit. It is uncertain as to why the green sandstones and mudstones of the KASH unit were not similarly altered and mineralised, although its different provenance may have been related to a change in the structural setting that changed the topography at surface and also the access to escaping fluids. During the formation of the Olympic Dam deposit, the continued extensional regime led to the tilting and collapse of large slabs of the 'bedded clastic facies' into the Olympic Breccia Complex, while the hydrothermal system remained active and to continued alteration and mineralisation, as on the margin of the KASH unit and within the deep KHEMQ fault slabs. Fluid activity appears to have continued sporadically, or as a reactivated event, after or during deposition of the 1575 to 1490 Ma Pandurra Formation.
Post-mineral Evolution
The Olympic Dam Breccia Complex and the Olympic Dam deposit were buried below the 400 to 2000 m thick Pandurra Formation of the ∼42 500 km2 Cariewerloo red bed basin. According to Giles, Betts and Lister, (2004), the Cariewerloo basin extension culminated in the separation of the North and South Australian cratons across a WNW-ESE line to the NE at ~1.5 Ga. These were followed by a series of tectonic and hydrothermal events.
that led to the structural and mineralogical reorganisation of the deposit, as follows:
• The inversion Cariewerloo Basin was followed by a long period of erosion and sedimentation. However, a scattering of dates evident in the uraninite of the deposit that are poorly constrained between 1300 and 1100 Ma, straddle the major regional ~1200 to 1160 Musgravian deformational event. These are suggested to be related to the sinistral fault dismemberment/displacement of the deposit that is evident on the main geological plan above. On a global scale, this activity coincided with the end of the break-up of the Columbia Super-continent and the initiation of assembly of Rodinia (Ehrig and Clark, 2020).
• There is some structural activity within the Olympic IOCG Province during the assembly of Rodinia between 1.1 and 0.9 Ga, including the possible uplit and exposure of the Pandurra Formation at ~1.1 Ga. The next significant event marked the onset of the break-up of that super-continent, namely the major ~825 Ma Gairdner Dyke Swarm large igneous province. Members of this swarm intrude the deposit with minor hydrothermal modifications and alteration related to their margins.
• During the Cryogenian period of the Neoproterozoic, the 750 to 700 Ma Sturtian and 660 to 636 Ma Marinoan glaciations levelled the topography above the Olympic Dam deposit and formed the Neoproterozoic unconformity surface on the Stuart Shelf. At Olympic Dam, this erosive event removed much of the Pandurra Formation, 'bedded clastic facies' and Gawler Range Volcanics that had protected and preserved the deposit for a billion years, and exposed the mineralisation. In the immediate deposit area, these eroded units were only preserved as down faulted remnants within the Olympic Dam Breccia Complex. However, this glacial levelling and exposure of the deposit was accompanied by deposition of a thick glacial and then shelf sequence, which continued to protect the deposit from further erosion to the present. The unconformity is only underlain by a thin palaeoregolith.
• As detailed above in the Mineralisation section, a significant period of U mineral formation occurred over the interval from 0.7 to 0.5 Ga, broadly consistent with the occurrence of non-euhedral uraninite that is part of a uranium assemblage that differs from those dated at ~1950 Ma. This second, younger assemblage is interpreted to imply widespread concomitant modification of precursor mineralisation, and possibly growth of new, uranium and sulphide minerals. This may be related to the unconformably overlying shelf sediment sequence that directly overlies the deposit, and/or the onset of the ~515 to 485 Ma Delamerian Orogeny during the assembly of the Gondwana block of the transient Pannotia supercontinent. Activity at the unconformity is indicated by alteration and sulphide mineralisation described above in paragraph vii). of the Carbonate Minerals section of the ALTERATION division.
• To the east, this Neoproterozoic to Phanerozoic cover sequence passes into the thicker, north-south oriented major 870 to 500 Ma Adelaide Rift Complex. This rift complex opened along the Palaeoproterozoic suture and separated the Mawson Craton from the Curnamona Province to the east. Later, during the Cretaceous, the separation of Antarctica resulted in the Australian segment of the Mawson Craton becoming the present Gawler Craton. In this sense, the location of the Olympic Dam deposit and the Olympic IOCG Province has progressed from near a cratonic margin during the Palaeoproterozoic, to intra-continental in the Late Palaeoproterozoic to the Early Neoproterozoic, to craton margin again from the Mid-Neoproterozoic.
RESERVES and RESOURCES
At the end of 1989, after commencing mining operations in mid-1988, reported resources and reserves (Reeve et al., 1990) amounted to:
Measured + indicated resource = 450 Mt @ 2.5% Cu, 0.6 g/t Au, 6.0 g/t Ag, 0.8 kg/tonne U3O8,
Inferred resource = 2000 Mt @ 1.6% Cu, 0.6 g/t Au, 3.5 g/t Ag, 0.6 kg/tonne U3O8,
Proved reserve = 13 Mt @ 3.0% Cu, 0.3 g/t Au, 10.2 g/t Ag, 1.1 kg/tonne U3O8,
Proved gold reserve = 2.3 Mt @ 1.6% Cu, 3.6 g/t Au, 2.9 g/t Ag, 0.3 kg/tonne U3O8.
At December 2004, published (BHP Billiton, 2005) reserves and resources were:
Proved+probable reserves totalled 761 Mt @ 1.5% Cu, 0.5 g/t Au, 0.5 kg/tonne U3O8,
within a total resource of 3810 Mt @ 1.1% Cu, 0.5 g/t Au, 0.4 kg/tonne U3O8.
At 30 June 2012, the published resources (BHP Billiton, September, 2012) amounted to:
Measured resource = 1474 Mt @ 1.03% Cu, 0.35 g/t Au, 1.95 g/t Ag, 0.30 kg/tonne U3O8,
Indicated resource = 4843 Mt @ 0.84% Cu, 0.34 g/t Au, 1.50 g/t Ag, 0.27 kg/tonne U3O8,
Inferred resource = 3259 Mt @ 0.70% Cu, 0.25 g/t Au, 0.98 g/t Ag, 0.23 kg/tonne U3O8,
Total resource = 9576 Mt @ 0.82% Cu, 0.31 g/t Au, 1.39 g/t Ag, 0.26 kg/tonne U3O8.
This resource includes a total proved + probable reserve of:
629 Mt @ 1.76% Cu, 0.73 g/t Au, 3.36 g/t Ag, 0.57 kg/tonne U3O8.
At the same date, the separate non-sulphide gold resource was 364 Mt @ 0.75 g/t Au, comprising:
Measured resource = 73 Mt @ 0.85 g/t Au; Indicated resource = 255 Mt @ 0.73 g/t Au; Inferred resource = 36 Mt @ 0.70 g/t Au.
At 30 June 2015, the published resources (BHP Billiton Annual Report, 2015) amounted to:
Measured resource = 1.330 Gt @ 0.96% Cu, 0.40 g/t Au, 2.0 g/t Ag, 0.29 kg/tonne U3O8,
Indicated resource = 4.610 Gt @ 0.79% Cu, 0.32 g/t Au, 1.0 g/t Ag, 0.24 kg/tonne U3O8,
Inferred resource = 4.120 Gt @ 0.71% Cu, 0.24 g/t Au, 1.0 g/t Ag, 0.25 kg/tonne U3O8,
Total resource = 10.060 Gt @ 0.78% Cu, 0.30 g/t Au, 1.0 g/t Ag, 0.25 kg/tonne U3O8.
This resource includes a total proved + probable reserve of:
484 Mt @ 1.95% Cu, 0.74 g/t Au, 4.0 g/t Ag, 0.59 kg/tonne U3O8.
Stockpile - 44 Mt @ 0.99% Cu, 0.51 g/t Au, 2.0 g/t Ag, 0.37 kg/tonne U3O8.
At 30 June 2015, a separate non-sulphide gold resource was 283 Mt @ 0.84 g/t Au, which was not reported in 2015.
At 30 June 2017, the published resources (BHP Annual Report, 2017) amounted to:
Measured resource = 1.460 Gt @ 0.96% Cu, 0.41 g/t Au, 2.0 g/t Ag, 0.30 kg/tonne U3O8,
Indicated resource = 4.680 Gt @ 0.79% Cu, 0.34 g/t Au, 1.0 g/t Ag, 0.25 kg/tonne U3O8,
Inferred resource = 3.920 Gt @ 0.71% Cu, 0.28 g/t Au, 1.0 g/t Ag, 0.24 kg/tonne U3O8,
Total resource = 10.100 Gt @ 0.78% Cu, 0.33 g/t Au, 1.0 g/t Ag, 0.25 kg/tonne U3O8.
This resource includes a total proved + probable reserve of:
508 Mt @ 1.99% Cu, 0.72 g/t Au, 4.0 g/t Ag, 0.58 kg/tonne U3O8.
Low grade Stockpile - 37 Mt @ 1.13% Cu, 0.51 g/t Au, 3.0 g/t Ag, 0.36 kg/tonne U3O8.
At 30 June 2022, the published resources (BHP Annual Report, 2022) amounted to:
Open Pit
Measured resource = 3.300 Gt @ 0.58% Cu, 0.32 g/t Au, 1.0 g/t Ag, 0.19 kg/tonne U3O8,
Indicated resource = 3.220 Gt @ 0.56% Cu, 0.24 g/t Au, 1.0 g/t Ag, 0.19 kg/tonne U3O8,
Inferred resource = 3.070 Gt @ 0.58% Cu, 0.23 g/t Au, 1.0 g/t Ag, 0.20 kg/tonne U3O8,
Total resource = 9.950 Gt @ 0.57% Cu, 0.26 g/t Au, 1.0 g/t Ag, 0.19 kg/tonne U3O8.
Underground
Measured resource = 0.800 Gt @ 1.55% Cu, 0.61 g/t Au, 3.0 g/t Ag, 0.46 kg/tonne U3O8,
Indicated resource = 0.750 Gt @ 1.42% Cu, 0.53 g/t Au, 3.0 g/t Ag, 0.43 kg/tonne U3O8,
Inferred resource = 0.18 Gt @ 1.40% Cu, 0.61 g/t Au, 3.0 g/t Ag, 0.39 kg/tonne U3O8,
Total resource = 1.730 Gt @ 1.48% Cu, 0.58 g/t Au, 3.0 g/t Ag, 0.44 kg/tonne U3O8.
TOTAL Underground + Open Pit Resource = 11.680 Gt @ 0.70% Cu, 0.31 g/t Au, 1.3 g/t Ag, 0.23 kg/tonne U3O8.
This resource includes a total proved + probable underground reserve of:
585 Mt @ 1.82% Cu, 0.67 g/t Au, 5.0 g/t Ag, 0.56 kg/tonne U3O8, plus
Low grade Stockpile - 42 Mt @ 0.81% Cu, 0.33 g/t Au, 2.0 g/t Ag, 0.27 kg/tonne U3O8.
Annual Production:
2005-06 totalled 204 300 tonnes of Cu, 3.34 t Au, 27.49 t Ag, 3936 tonnes U3O8.
2008-09 totalled 194 100 tonnes of Cu, 3.36 t Au, 29.14 t Ag, 4007 tonnes U3O8.
2011-12 totalled 192 600 tonnes of Cu, 3.66 t Au, 28.21 t Ag, 3885 tonnes U3O8.
2014-15 totalled 124 500 tonnes of Cu, 3.26 t Au, 22.52 t Ag, 3144 tonnes U3O8.
2020-21 totalled 205 300 tonnes of Cu, 4.54 t Au, 22.2 t Ag, 3267 tonnes U3O8.
2021-22 totalled 138 400 tonnes of Cu, 3.72 t Au, 23.1 t Ag, 2375 tonnes U3O8.
The mine is owned and operated by a subsidiary of BHP Ltd.
The most recent source geological information used to prepare this decription was dated: 2024.
Record last updated: 2/3/2024
This description is a summary from published sources, the chief of which are listed below. © Copyright Porter GeoConsultancy Pty Ltd. Unauthorised copying, reproduction, storage or dissemination prohibited.
Olympic Dam
|
|
Apukhtina, O.B, Kamenetsky, V.S., Ehrig, K., Kamenetsky, M.B., Maas, R., Thompson, J., McPhie, Cristiana, J., Ciobanu, L. and Cook, N.J., 2017 - Early, deep magnetite-fluorapatite mineralization at the Olympic Dam Cu-U-Au-Ag Deposit, South Australia: in Econ. Geol. v.112, pp. 1531-1542.
|
Apukhtina, O.B., Ehrig, K., Kamenetsky, V.S., Kamenetsky, M.B., Goemann, K., Maas, R., McPhie, J., Cook, N.J. and Ciobanu, C.L., 2020 - Carbonates at the supergiant Olympic Dam Cu-U-Au-Ag deposit, South Australia. Part 1: Distribution, textures, associations and stable isotope (C, O) signatures: in Ore Geology Reviews v.126, 17p. doi.org/10.1016/j.oregeorev.2020.103775.
|
Belpario A and Freeman H 2004 - Common geological characteristics of Prominent Hill and Olympic Dam - Implications for iron oxide copper-gold exploration models : in Hi Tech and World Competitive Mineral Success Stories Around the Pacific Rim, Proc. Pacrim 2004 Conference, Adelaide, 19-22 September, 2004, AusIMM, Melbourne pp 115-125
|
Blein, O., Corriveau, L., Montreuil, J.-F., Ehrig, K., Fabris, A., Reid, A. and Pal, D., 2022 - Geochemical signatures of metasomatic ore systems hosting IOCG, IOA, albitite-hosted uranium and affiliated deposits: A tool for process studies and mineral exploration,: in Corriveau, L., Potter, E.G. and Mumin, A.H., (Eds.), 2022 Mineral systems with iron oxide-copper-gold (IOCG) and affiliated deposits, Geological Association of Canada, Special Paper 52, pp. 263-298.
|
Cherry, A.R., Ehrig, K., Kamenetsky, V.S., Ehrig, K. and McPhie, J., 2017 - Constraints on the setting, timing and incorporation of surficial lithologies into the Olympic Dam Breccia Complex: in Poster 1p.
|
Cherry, A.R., Ehrig, K., Kamenetsky, V.S., McPhie, J., Crowley, J.L. and Kamenetsky, M.B., 2018 - Precise geochronological constraints on the origin, setting and incorporation of ca. 1.59 Ga surficial facies into the Olympic Dam Breccia Complex, South Australia: in Precambrian Research v.315, pp. 162-178. doi.org/10.1016/ j.precamres.2018.07.01.
|
Cherry, A.R., McPhie, J., Kamenetsky, V.S., Ehrig, K., Keeling, J.L., Kamenetsky, M.B., Meffre, S. and Apukhtina, O.B., 2017 - Linking Olympic Dam and the Cariewerloo Basin: Was a sedimentary basin involved in formation of the worlds largest uranium deposit?: in Precambrian Research v.300, pp. 168-180.
|
Ciobanu, C.L., Cook, N.J. and Ehrig, K., 2016 - Ore minerals down to the nanoscale: Cu-(Fe)-sulphides from the iron oxide copper gold deposit at Olympic Dam, South Australia: in Ore Geology Reviews v.81, pp. 1218-1235.
|
Ciobanu, C.L., Verdugo-Ihl, M.R., Slattery, A., Cook, N.J., Ehrig, K., Courtney-Davies, L. and Wade, B.P., 2019 - Silician Magnetite: Si-Fe-Nanoprecipitates and Other Mineral Inclusions in Magnetite from the Olympic Dam Deposit, South Australia: in Minerals (MDPI) v.9, 35p. doi:10.3390/min9050311.
|
Ciobanu, C.L., Wade, B.P., Cook, N.J., Schmidt Mumm, A. and Giles, D., 2013 - Uranium-bearing hematite from the Olympic Dam Cu-U-Au deposit, South Australia: A geochemical tracer and reconnaissance Pb-Pb geochronometer: in Precambrian Research v.238, pp. 129-147
|
Clark, J.M. and Ehrig, K., 2019 - What controls high-grade copper mineralisation at Olympic Dam?: in Mining Geology 2019, Perth, Western Australia on 25-26 November 2019, The Australasian Institute of Mining and Metallurgy: Melbourne, Proceedings, pp. 222-236.
|
Corriveau, L., Montreuil, J.-F., Blein, O., Ehrig, K., Potter, E.G., Fabris. A. and Clark, J., 2022 - Mineral systems with IOCG and affiliated deposits: Part 2 - geochemical footprints: in Corriveau, L., Potter, E.G. and Mumin, A.H., (Eds.), 2022 Mineral systems with iron oxide-copper-gold (IOCG) and affiliated deposits, Geological Association of Canada, Special Paper 52, pp. 159-204.
|
Corriveau, L., Montreuil, J.-F., Potter, E.G, Blein, O. and De Toni, A.F., 2022 - Mineral systems with IOCG and affiliated deposits: Part 3 - metal pathways and ore deposit model,: in Corriveau, L., Potter, E.G. and Mumin, A.H., (Eds.), 2022 Mineral systems with iron oxide-copper-gold (IOCG) and affiliated deposits, Geological Association of Canada, Special Paper 52, pp. 205-245.
|
Corriveau, L., Montreuil, J.-F., Potter, E.G., Ehrig, K., Clark, J.M., Mumin, A.H. and Williams, P.J., 2022 - Mineral systems with IOCG and affiliated deposits: Part 1 - metasomatic footprints of alteration facies: in Corriveau, L., Potter, E.G. and Mumin, A.H., (Eds.), 2022 Mineral systems with iron oxide-copper-gold (IOCG) and affiliated deposits, Geological Association of Canada, Special Paper 52, pp. 113-158.
|
Courtney-Davies, L., Ciobanu, C.L., Tapster, S.R., Cook, N.J., Ehrig, K., Crowley, J.L., Verdugo-Ihl, M.R., Wade, B.P. and Condon, D.J., 2020 - Opening the magmatic-hydrothermal window: high-precision U-Pb geochronology of the Mesoproterozoic Olympic Dam Cu-U-Au-Ag Deposit, South Australia: in Econ. Geol. v.115, pp. 1855-1870.
|
Courtney-Davies, L., Ciobanu, C.L., Verdugo-Ihl, M.R., Cook, N.J., Ehrig, K.J., Wade, B.P., Zhu, Z.-Y. and Kamenetsky, V.S., - ~1760 Ma magnetite-bearing protoliths in the Olympic Dam deposit, South Australia: Implications for ore genesis and regional metallogeny: in Ore Geology Reviews v.118, pp. 1-13. doi.org/10.1016/j.oregeorev.2020.103337.
|
Courtney-Davies, L., Ciobanu, C.L., Verdugo-Ihla, M.R., Dmitrijeva, M., Cook, N.J., Ehrig, K. and Wade, B.P., 2019 - Hematite geochemistry and geochronology resolve genetic and temporal links among iron-oxide copper gold systems, Olympic Dam district, South Australia: in Precambrian Research v.335, doi.org/10.1016/j.precamres.2019.105480.
|
Curtis, S., Wade, C. and Reid, A., 2018 - Sedimentary basin formation associated with a silicic large igneous province: stratigraphy and provenance of the Mesoproterozoic Roopena Basin, Gawler Range Volcanics: in Australian J. of Earth Sciences v.65, pp. 447-463.
|
Dmitrijeva, M., Ehrig, K.J., Ciobanu, C.L., Cook, N.J., Verdugo-Ihl, M.R. and Metcalfe, A.V., 2019 - Defining IOCG signatures through compositional data analysis: A case study of lithogeochemical zoning from the Olympic Dam deposit, South Australia: in Ore Geology Reviews v.105, pp. 86-101.
|
Drummond B, Lyons P, Goleby B and Jones J, 2006 - Constraining models of the tectonic setting of the giant Olympic Dam iron oxide-copper-gold deposit, South Australia, using deep seismic reflection data: in Tectonophysics v420 pp 91-103
|
Ehrig K, 2010 - Olympic Dam: 35 years since discovery, 2.3 million metres of diamond core and new ideas - Abstract: in Giant Ore Deposits Down Under 13th Quadrennial IAGOD Symposium, Adelaide, South Australia, 6-9 April, 2010, Symposium Proceedings, pp. 9-10
|
Ehrig, K. and Clark, J., 2020 - Unravelling the geology of Olympic Dam: in Ore Deposit Hub - https://www.youtube.com/watch?v=RCwR9fPfcEk, Video - 1 hr 40 min, 29 p. of slides.
|
Ehrig, K., 2022 - Olympic Dam Geology - an overview: in NExUS-PD: South Australian IOCG Mineral Systems Workshop, Tonsley, South Australia, 29-30 September, 2022. Presentation compilation, 34p.
|
Ehrig, K., Kamenetsky, V.S., McPhie, J., Cook, N.J. and Ciobanu, C.L., 2017 - Olympic Dam iron oxide Cu-U-Au-Ag deposit: in Phillips, G.N., (Ed.), 2017 - Australian Ore Deposits, The Australasian Institute of Mining and Metallurgy, Mono 32, pp. 601-610.
|
Ehrig, K., Kamenetsky, V.S., McPhie, J., Macmillan, E., Thompson, J., Kamenetsky, M. and Maas, R., 2021 - Staged formation of the supergiant Olympic Dam uranium deposit, Australia: in Geology v.49, pp. 1312-1316. doi.org/10.1130/G48930.1.
|
Ehrig, K., McPhie, J. and Kamenetsky, V., 2012 - Geology and Mineralogical Zonation of the Olympic Dam Iron Oxide Cu-U-Au-Ag Deposit, South Australia: in Hedenquist, J.W., Harris, M. and Camus, F., 2012 - Geology and Genesis of Major Copper Deposits and Districts of the World - A tribute to Richard H Sillitoe, Society of Economic Geologists Special Publication 16, pp. 237-267.
|
Ehrig, K., Schijns, H., Townsend, J., Haddow, D. and Shawcross,M., 2023 - Seismic velocity (Vp) vs alteration mineralogy in the Olympic Dam deposit: in Breaking New Ground, 4th AEGC Conference, 13-18 March 2023, Brisbane, Australia, Proceedings, 10p.
|
Esdale, D., Pridmore, D.F., Coggon, J., Muir, P., Williams, P. and Fritz, F., 2003 - The Olympic Dam copper-uranium-gold-silver rare earth element deposit, South Australia: A geophysical case history: in ASEG Extended Abstracts, 2003;3, pp. 147-168. doi.org/10.1071/ASEGSpec12_13.
|
Fabris, A., 2022 - Geochemical characteristics of IOCG deposits from the Olympic Copper-Gold Province, South Australia: in Corriveau, L., Potter, E.G. and Mumin, A.H., (Eds.), 2022 Mineral systems with iron oxide coppergold (IOCG) and affiliated deposits Geological Association of Canada, Special Paper 52, pp. 247-262.
|
Ferris G M, Schwartz M P and Heithersay P 2002 - The geological framework, distribution and controls of Fe-Oxide Cu-Au mineralisation in the Gawler Craton, South Australia: Part 1 - Geological and tectonic framework: in Porter T M 2002 Hydrothermal Iron Oxide Copper-Gold and Related Deposits: A Global Perspective PGC Publishing, Adelaide v2 pp 9-31
|
Haynes D W, 2000 - Iron Oxide Copper (-Gold) Deposits: Their Position in the Ore Deposit Spectrum and Modes of Origin: in Porter T M (Ed), 2000 Hydrothermal Iron Oxide Copper-Gold & Related Deposits: A Global Perspective PGC Publishing, Adelaide v.1 pp. 71-90
|
Haynes D W, Cross K C, Bills R T, Reed M H 1995 - Olympic Dam ore genesis: a fluid mixing model: in Econ. Geol. v90 pp 281-307
|
Hayward N and Skirrow R, 2010 - Geodynamic Setting and Controls on Iron Oxide Cu-Au (±U) Ore in the Gawler Craton, South Australia: in Porter T M, (Ed), 2010 Hydrothermal Iron Oxide Copper-Gold and Related Deposits: A Global Perspective PGC Publishing, Adelaide v.3 pp. 119-146
|
Heinson, G., Didana, Y., Soeffky, P., Thiel, S. and Wise, T., 2018 - The crustal geophysical signature of a world-class magmatic mineral system: in Scientific Reports 2018:8, DOI:10.1038/s41598-018-29016-2.
|
Heinson, G.S., Direen, N.G. and Gill, R.G., 2006 - Magnetotelluric evidence for a deep-crustal mineralizing system beneath the Olympic Dam iron oxide copper-gold deposit, southern Australia: in Geology v.34, pp. 573-576.
|
Hitzman M W and Valenta R K, 2005 - Uranium in iron oxide-copper-gold (IOCG) systems: in Econ. Geol. v100 pp 1657-1661
|
Huang, Q., Kamenetsky, V.S., Ehrig, K., McPhie, J., Kamenetsky, M., Cross, K., Meffre, S., Agangi, A, Chambefort, I., Direen, N.G., Maas, R. and Apukhtina, O., 2016 - Olivine-phyric basalt in the Mesoproterozoic Gawler silicic large igneous province, South Australia: Examples at the Olympic Dam Iron Oxide Cu-U-Au-Ag deposit and other localities: in Precambrian Research v.281, pp. 185-199.
|
Huang, Q., Kamenetsky, V.S., Ehrig, K., McPhie, J., Kamenetsky, M.B., Apukhtina, O.B. and Chambefort, I., 2017 - Effects of hydrothermal alteration on mafic lithologies at the Olympic Dam Cu-U-Au-Ag deposit: in Precambrian Research v.292, pp. 305-322.
|
Huang, Q., Kamenetsky, V.S., McPhie, J., Ehrig, K., Meffre, S., Maas, R., Thompson, J., Kamenetsky, M., Chambefort, I., Apukhtina, O. and Hu, Y., 2015 - Neoproterozoic (ca. 820-830 Ma) mafic dykes at Olympic Dam, South Australia: Links with the Gairdner Large Igneous Province: in Precambrian Research v.271, pp. 160-172.
|
Jagodzinski, E.A., Crowley, J.L., Reid, A.J., Wade, C.E. and Bockmann, M.J., 2021 - High-precision CA-TIMS dating of the Hiltaba Suite, Gawler Craton: in Department for Energy and Mining, South Australia, Adelaide, Report Book 2021/00002, 63p.
|
Johnson J P, Cross K C 1995 - U-Pb geochronological constraints on the genesis of the Olympic Dam Cu-U-Au-Ag deposit, South Australia: in Econ. Geol. v.90 pp 1046-1063
|
Knutson J, Donnelly T H, Eadington P J and Tonkin D G, 1992 - Hydrothermal alteration of middle Proterozoic basalts, Stuart Shelf, South Australia; a possible source for Cu mineralization : in Econ. Geol. v.87 pp. 1054-1077
|
Kontonikas-Charos, A., Ciobanu, C.L., Cook, N.J., Ehrig, K., Ismail, R., Krneta, S. and Basak, A., 2018 - Feldspar mineralogy and rare-earth element (re)mobilization in iron-oxide copper gold systems from South Australia: a nanoscale study: in Mineralogical Magazine v.82 (S1), pp. S173-S197.
|
Kontonikas-Charos, A., Ciobanu, C.L., Cook, N.J., Ehrig, K., Krneta, S. and Kamenetsky, V.S., 2017 - Feldspar evolution in the Roxby Downs Granite, host to Fe-oxide Cu-Au-(U) mineralisation at Olympic Dam, South Australia: in Ore Geology Reviews v.80, pp. 838-859.
|
Krneta, S., Ciobanub, C.L., Cook, N.J., Ehrig, K. and Kontonikas-Charos, A., 2016 - Apatite at Olympic Dam, South Australia: A petrogenetic tool: in Lithos v.262, pp. 470-485.
|
Maas, R., Apukhtina, O.B., Kamenetsky, V.S., Ehrig, K., Sprung, P. and Munker, C., 2022 - Carbonates at the supergiant Olypmic Dam Cu-U-Au-Ag deposit, South Australia. Part 2: Sm-Nd, Lu-Hf and Sr-Pb isotope constraints on the chronology of carbonate deposition: in Ore Geology Reviews v.140, 16p. doi.org/10.1016/j.oregeorev.2020.103745.
|
Mauger, A.J., Ehrig, K., Kontonikas-Charos, A., Ciobanu, C.L., Cook, N.J. and Kamenetsky, V.S., 2016 - Alteration at the Olympic Dam IOCG-U deposit: insights into distal to proximal feldspar and phyllosilicate chemistry from infrared reflectance spectroscopy: in Australian J. of Earth Sciences v.63, pp. 959-97.
|
McPhie J, Kamenetsky V, Allen S, Ehrig K, Agangi A and Bath A, 2011 - The fluorine link between a supergiant ore deposit and a silicic large igneous province: in Geology v.39 pp. 1003-1006
|
McPhie J, Kamenetsky V, Chambefort I, Ehrig K and Green N, 2010 - The origin of Olympic Dam: A revolutionary new view - Abstract: in Giant Ore Deposits Down Under 13th Quadrennial IAGOD Symposium, Adelaide, South Australia, 6-9 April, 2010, Symposium Proceedings, pp. 76-77
|
McPhie, J., Kamenetsky, V., Chambefort, I., Ehrig, K. and Green, N., 2011 - Origin of the supergiant Olympic Dam Cu-U-Au-Ag deposit, South Australia: Was a sedimentary basin involved ?: in Geology v.39 pp. 795-798
|
McPhie, J., Orth, K., Kamenetsky, V.S., Kamenetsky, M. and Ehrig, K., 2016 - Characteristics, origin and significance of Mesoproterozoic bedded clastic facies at the Olympic Dam Cu-U-Au-Ag deposit, South Australia: in Precambrian Research v.276, pp. 85-100.
|
Oreskes N and Hitzman M W, 1993 - A model for the origin of Olympic Dam-type deposits: in Kirkham R V, Sinclair W D, Thorpe R I, Duke J M, (Eds) 1993 Mineral Deposit Modelling Geological Association of Canada Special Paper 40 pp. 615-633
|
Oreskes N, Einaudi M T 1990 - Origin of rare earth element-enriched Hematite breccias at the Olympic Dam Cu-U-Au-Ag deposit, Roxby Downs, South Australia: in Econ. Geol. v85 pp 1-28
|
Oreskes, N. and Einaudi, M.T., 1992 - Origin of hydrothermal fluids at Olympic Dam: preliminary results from fluid inclusions and stable isotopes: in Econ. Geol. v.87, pp. 64-90.
|
Prado, E.M.G., de Souza Filho, C.R. and Carranza, E.J.M., 2023 - Ore Grade Estimation from Hyperspectral Data Using Convolutional Neural Networks: A Case Study at the Olympic Dam Iron Oxide Copper-Gold Deposit, Australia: in Econ. Geol. v.118, pp. 1899-1921. doi: 10.5382/econgeo.5023.
|
Reeve J S, Cross K C, Smith R N, Oreskes N 1990 - Olympic Dam copper-uranium-gold-silver deposit: in Hughes F E (Ed), Geology of the Mineral Deposits of Australia and Papua New Guinea The AusIMM, Melbourne v2 pp 1009-1035.
|
Reynolds L J 2000 - Geology of the Olympic Dam Cu-U-Au-Ag-REE deposit: in Porter T M (Ed), 2000 Hydrothermal Iron Oxide Copper-Gold & Related Deposits: A Global Perspective PGC Publishing, Adelaide v1 pp. 93-104
|
Roberts, D.E. and Hudson, G.R.T., 1983 - The Olympic Dam Copper-Uranium-Gold Deposit, Boxby Downs, South Australia: in Econ. Geol. v.78, pp. 799-822.
|
Schlegel, T.U., Wagner, T. and Fusswinkel, T., 2020 - Fluorite as indicator mineral in iron oxide-copper-gold systems: Explaining the IOCG deposit diversity: in Chemical Geology v.548, doi.org/10.1016/j.chemgeo.2020.119674.
|
Skirrow R G, Bastrakov E, Raymond O L, Davidson G and Heithersay P, 2002 - The geological framework, distribution and controls of Fe-Oxide Cu-Au mineralisation in the Gawler Craton, South Australia: Part 2 - Alteration and mineralisation: in Porter T M (Ed.), 2002 Hydrothermal Iron Oxide Copper-Gold and Related Deposits: A Global Perspective, PGC Publishing, Adelaide v2 pp 33-47
|
Skirrow, R. G., van der Wielen, S. E., Champion, D. C., Czarnota, K. and Thiel, S., 2018 - Lithospheric Architecture and Mantle Metasomatism Linked to Iron Oxide Cu-Au Ore Formation: Multidisciplinary Evidence from the Olympic Dam Region, South Australia: in AGU100 Advancing Earth and Space Science, Geochemistry, Geophysics, Geosystems, 19, doi.org/10.1029/ 2018GC007561 pp. 2673-2705.
|
Skirrow, R.G., 2022 - Hematite-group IOCG ±U deposits: an update on their tectonic settings, hydrothermal characteristics, and Cu-Au-U mineralizing processes: in Corriveau, L., Potter, E.G. and Mumin, A.H., (Eds.), 2022 Mineral systems with iron oxide-copper-gold (IOCG) and affiliated deposits, Geological Association of Canada, Special Paper 52, pp. 27-51.
|
Smith R J, 2002 - Geophysics of Iron Oxide Copper-Gold Deposits: in Porter T M (Ed.), 2002 Hydrothermal Iron Oxide Copper-Gold and Related Deposits: A Global Perspective, PGC Publishing, Adelaide v.2 pp. 357-367
|
Smith R N 1993 - Olympic Dam: some developments in geological understanding over nearly two decades: in The AusIMM Centenary Conference, Adelaide, 30 March-4 April, 1993 AusIMM Publication Series 2/93 pp 113-118
|
Smith, M.P., Moore, K., Kavecsanszki, D., Finch, A.A., Kynicky, J. and Wall, F., 2016 - From mantle to critical zone: A review of large and giant sized deposits of the rare earth elements: in Geoscience Frontiers v.7, pp. 315-334.
|
Tappert, M.C., Rivard, B., Giles, D., Tappert, R. and Mauger, A., 2013 - The mineral chemistry, near-infrared, and mid-infrared reflectance spectroscopy of phengite from the Olympic Dam IOCG deposit, South Australia: in Ore Geology Reviews v.53, pp. 26-38,
|
Townsend, J., Schijns, H., Ehrig, K., Haddow, D. and Shawcross,M., 2023 - The First Sparse 3D Hardrock Seismic Survey at Olympic Dam: in Breaking New Ground, 4th AEGC Conference, 13-18 March 2023, Brisbane, Australia, Proceedings, 8p.
|
Verdugo-Ihl, M.R., Ciobanu, C.L., Cook, N.J., Ehrig, K.J. and Courtney-Davies, L., 2020 - Defining early stages of IOCG systems: evidence from iron oxides in the outer shell of the Olympic Dam deposit, South Australia: in Mineralium Deposita v.55, pp. 429-452.
|
Verdugo-Ihl, M.R., Ciobanub, C.L., Cook, N.J., Ehrig, K., Courtney-Davies, L. and Gilbert, S., 2017 - Textures and U-W-Sn-Mo signatures in hematite from the Olympic Dam Cu-U-Au-Ag deposit, South Australia: Defining the archetype for IOCG deposits: in Ore Geology Reviews in press pp. NA.
|
Wade, C.E., Payne, J.L., Barovich, K.M. and Reid, A.J., 2019 - Heterogeneity of the sub-continental lithospheric mantle and non-juvenile mantle additions to a Proterozoic silicic large igneous province: in Lithos v.340-341, pp. 87-107.
|
Wade, C.E., Reid, A.J., Payne, J.L. and Barovich, K.M., 2019 - The link is in the geochemistry: how we know a metasomatised mantle underlies the mineral systems of the Gawler Craton: in Mesa Journal v.90, pp. 42-47.
|
Widdup H, Fouet T, Hodgkison J, McCuaig T C and Miller J 2004 - A three dimensional structural interpretation of the Olympic Dam deposit - Implications for mine planning and exploration: in Hi Tech and World Competitive Mineral Success Stories Around the Pacific Rim, Proc. Pacrim 2004 Conference, Adelaide, 19-22 September, 2004, AusIMM, Melbourne pp 417-426
|
Williams P J, Barton M D, Johnson D A, Fontbote L, de Haller A, Mark G, Oliver N H S and Marschik R, 2005 - Iron oxide copper-gold deposits: Geology, space-time distribution and possible modes of origin: in Hedenquist J W, Thompson J F H, Goldfarb R J and Richards J P (Eds.), 2005 Economic Geology 100th Anniversary Volume, Society of Economic Geologists, Denver, pp 371-405
|
Williams, P. J., Kendrick, M.A. and Xavier, R.P., 2010 - Sources of Ore Fluid Components in IOCG Deposits: in Porter T M, (Ed), 2010 Hydrothermal Iron Oxide Copper-Gold and Related Deposits: A Global Perspective PGC Publishing, Adelaide v.3, pp. 107-116.
|
Williams, P.J. and Pollard, P.J., 2003 - Australian Proterozoic Iron Oxide-Cu-Au Deposits: An Overview with New Metallogenic and Exploration Data from the Cloncurry District, Northwest Queensland: in Exploration & Mining Geology, CIM v.10, No. 3, pp. 191-213.
|
Zhu, Z., 2016 - Gold in iron oxide copper-gold deposits: in Ore Geology Reviews v.72, pp. 37-42.
|
Porter GeoConsultancy Pty Ltd (PorterGeo) provides access to this database at no charge. It is largely based on scientific papers and reports in the public domain, and was current when the sources consulted were published. While PorterGeo endeavour to ensure the information was accurate at the time of compilation and subsequent updating, PorterGeo, its employees and servants: i). do not warrant, or make any representation regarding the use, or results of the use of the information contained herein as to its correctness, accuracy, currency, or otherwise; and ii). expressly disclaim all liability or responsibility to any person using the information or conclusions contained herein.
|
Top | Search Again | PGC Home | Terms & Conditions
|
|